![]()
An artist's impression of the ELT
| |
Alternative names | ELT |
---|---|
Observatory | Cerro Armazones Observatory |
Location(s) | Cerro Armazones, Chile |
Coordinates | 24°35′52″S 70°11′46″WCoordinates: 24°35′52″S 70°11′46″W |
Organization | European Southern Observatory |
Altitude | 3,046 m (9,993 ft) |
Observing time | 320 nights per year |
Built | 19 June 2014 -- |
First light | 2024 |
Telescope style | Extremely large telescope Infrared telescope Nasmyth telescope Optical telescope |
Diameter | 39.3 m (128 ft 11 in) |
Secondary diameter | 4.09 m (13 ft 5 in) |
Tertiary diameter | 3.75 m (12 ft 4 in) |
Angular resolution | 0.005 arcsecond |
Collecting area | 978 m2 (10,530 sq ft) |
Focal length | 743.4 m (2,439 ft 0 in) |
Mounting | Nasmyth telescope |
Enclosure | Dome |
Website | www![]() |
The Extremely Large Telescope (ELT) is an astronomical observatory and the world's largest optical/near-infrared extremely large telescope now under construction. Part of the European Southern Observatory (ESO) agency, it is located on top of Cerro Armazones in the Atacama Desert of northern Chile. The design consists of a reflecting telescope with a 39.3-metre-diameter (130-foot) segmented primary mirror and a 4.2 m (14 ft) diameter secondary mirror, and will be supported by adaptive optics, eight laser guide star units and multiple large science instruments. The observatory aims to gather 100 million times more light than the human eye, 13 times more light than the largest optical telescopes existing in 2014, and be able to correct for atmospheric distortion. It has around 256 times the light gathering area of the Hubble Space Telescope and, according to the ELT's specifications, would provide images 16 times sharper than those from Hubble. The project was originally called the European Extremely Large Telescope (E-ELT), but the name was shortened in 2017.
The ELT is intended to vastly advance astrophysical knowledge by enabling detailed studies of planets around other stars, the first galaxies in the Universe, supermassive black holes, and the nature of the Universe's dark sector, and to detect water and organic molecules in protoplanetary disks around other stars. The facility is expected to take 11 years to construct.
On 11 June 2012, the ESO Council approved the ELT programme's plans to begin civil works at the telescope site, with construction of the telescope itself pending final agreement with governments of some member states. Construction work on the ELT site started in June 2014. By December 2014, ESO had secured over 90% of the total funding and authorized construction of the telescope to start, which will cost around one billion euros for the first construction phase. The first stone of the telescope was ceremonially laid on 26 May 2017, initiating the construction of the dome’s main structure and telescope, with first light being planned for 2024.
History
On 26 April 2010, the European Southern Observatory (ESO) Council selected Cerro Armazones, Chile, as the baseline site for the planned ELT. Other sites that were under discussion included Cerro Macon, Salta, in Argentina; Roque de los Muchachos Observatory, on the Canary Islands; and sites in North Africa, Morocco, and Antarctica.
Early designs included a segmented primary mirror with a diameter of 42 metres (140 feet) and an area of about 1,300 m2
(14,000 sq ft), with a secondary mirror with a diameter of 5.9 m
(19 ft). However, in 2011 a proposal was put forward to reduce its size
by 13% to 978 m2, for a 39.3 m (130 ft) diameter primary mirror and a 4.2 m (14 ft) diameter secondary mirror.
It reduced projected costs from 1.275 billion to 1.055 billion euros
and should allow the telescope to be finished sooner. The smaller
secondary is a particularly important change; 4.2 m (14 ft) places it
within the capabilities of multiple manufacturers, and the lighter
mirror unit avoids the need for high-strength materials in the secondary
mirror support spider.
ESO Council meets at ESO headquarters in Garching, 2012.
ESO's Director General commented in a 2011 press release that "With
the new E-ELT design we can still satisfy the bold science goals and
also ensure that the construction can be completed in only 10–11 years."
The ESO Council endorsed the revised baseline design in June 2011 and
expected a construction proposal for approval in December 2011. Funding was subsequently included in the 2012 budget for initial work to begin in early 2012. The project received preliminary approval in June 2012. ESO approved the start of construction in December 2014, with over 90% funding of the nominal budget secured.
The design phase of the 5-mirror anastigmat
was fully funded within the ESO budget. With the 2011 changes in the
baseline design (such as a reduction in the size of the primary mirror
from 42 m to 39.3 m), in 2017 the construction cost was estimated to be
€1.15 billion (including first generation instruments). As of 2014, the start of operations was planned for 2024. Actual construction officially began in early 2017.
ELT foundation work, October 2018.
Planning
Cerro Armazones at night (2010)
ESO focused on the current design after a feasibility study concluded the proposed 100 m (328 ft) diameter, Overwhelmingly Large Telescope,
would cost €1.5 billion (£1 billion), and be too complex. Both current
fabrication technology and road transportation constraints limit single
mirrors to being roughly 8 m (26 ft) per piece. The next-largest
telescopes currently in use are the Keck Telescopes, the Gran Telescopio Canarias and the Southern African Large Telescope,
which each use small hexagonal mirrors fitted together to make a
composite mirror slightly over 10 m (33 ft) across. The ELT uses a
similar design, as well as techniques to work around atmospheric
distortion of incoming light, known as adaptive optics.
A 40-metre-class mirror will allow the study of the atmospheres of extrasolar planets. The ELT is the highest priority in the European planning activities for research infrastructures, such as the Astronet Science Vision and Infrastructure Roadmap and the ESFRI Roadmap.
The telescope underwent a Phase B study in 2014 that included
"contracts with industry to design and manufacture prototypes of key
elements like the primary mirror segments, the adaptive fourth mirror or
the mechanical structure (...) [and] concept studies for eight
instruments.”
Design
The ELT will use a novel design with a total of five mirrors. The first three mirrors are curved (non-spherical), and form a three mirror anastigmat
design for excellent image quality over the 10 arcminute field of view
(one third of the width of the full Moon). The fourth and fifth mirrors
are (almost) flat, and provide adaptive optics
correction for atmospheric distortions (mirror 4), and tip-tilt
correction for image stabilisation (mirror 5). The fourth and fifth
mirrors also send the light sideways to one of the Nasmyth focal stations at either side of the telescope structure, allowing multiple large instruments to be simultaneously mounted.
ELT mirror and sensors contracts
Primary mirror
The optical system of the ELT showing the location of the mirrors.
The surface of the 39-metre primary mirror will be composed of 798
hexagonal segments, each measuring approximately 1.4 metres across and
with 50 mm thickness. Each working day, two segments will be re-coated and replaced to ensure the mirror is always clean and highly reflective.
Edge sensors constantly measure the relative positions of the primary mirror segments and their neighbours. 2394 position actuators
(3 for each segment) use this information to support the system,
keeping the overall surface shape unchanged against deformations caused
by external factors such as wind, temperature changes or vibrations.
In January 2017, ESO awarded the contract for the fabrication of the 4608 edge sensors to the FAMES consortium, which is composed of Fogale and Micro-Epsilon. These sensors can measure relative positions to an accuracy of a few nanometres, the most accurate ever used in a telescope.
Cast of the first ELT main mirror segments.
In May 2017, ESO awarded two additional contracts. One was awarded to Schott AG
who will manufacture the blanks of the 798 segments, as well as an
additional 133 segments as part of a maintenance set, allowing for the
segments to be removed, replaced and cleaned on a rotating basis once
the ELT is in operation. The mirror will be cast from the same
low-expansion ceramic Zerodur as the existing Very Large Telescope mirrors in Chile.
The other contract was awarded to the French company, Safran Reosc, a subsidiary of Safran Electronics & Defense.
They will receive the mirror blanks from Schott, and polish one mirror
segment per day to meet the 7-year deadline. During this process, each
segment will be polished until it has no surface irregularity greater
than 7.5 nm RMS.
Afterwards, Safran Reosc will then mount, test, and complete all
optical testing before delivery. This is the second largest contract for
the ELT construction and the third-largest contract ESO has ever
signed.
The segment support system units for the primary mirror are designed and produced by CESA (Spain) and VDL
(the Netherlands). The contracts signed with ESO also include the
delivery of detailed and complete instructions and engineering drawings
for their production. Additionally, they include the development of the
procedures required to integrate the supports with the ELT glass
segments; to handle and transport the segment assemblies; and to operate
and maintain them.
Secondary mirror
This
sequence shows the still very hot blank of the ELT secondary mirror
being transported to Schott's annealing facility in Mainz, Germany.
Making the secondary mirror is a major challenge as it is highly
convex, and aspheric. It is also very large; at 4.2 metres in diameter
and weighing 3.5 tonnes, it will be largest secondary ever employed on a
telescope and the largest convex mirror ever produced.
In January 2017, ESO awarded a contract for the mirror blank to Schott AG, who will manufacture it of Zerodur.
Complex support cells are also necessary to ensure the flexible
secondary and tertiary mirrors retain their correct shape and position;
these support cells will be provided by SENER.
The pre-formed glass-ceramic blank of the secondary mirror will then be polished, and tested by Safran Reosc.
The mirror will be shaped and polished to a precision of 15 nanometres
(15 millionths of a millimetre) over the optical surface.
Tertiary mirror
The
3.8-metre concave tertiary mirror, also cast from Zerodur, will be an
unusual feature of the telescope. Most current large telescopes,
including the VLT and the NASA/ESA Hubble Space Telescope, use just two
curved mirrors to form an image. In these cases, a small, flat tertiary
mirror is sometimes introduced to divert the light to a convenient
focus. However, in the ELT the tertiary mirror also has a curved
surface, as the use of three mirrors delivers a better final image
quality over a larger field of view than would be possible with a
two-mirror design.
Quaternary mirror
The
2.4-metre quaternary mirror is a flat adaptive mirror, and only 2
millimetres thick. With up to 8000 actuators, the surface can be
readjusted at very high time frequencies. The deformable mirror will be the largest adaptive mirror ever made,
and consists of six component petals, control systems, and voice-coil
actuators. The image distortion caused by the turbulence of the Earth’s
atmosphere can be corrected in real time, as well as deformations caused
by the wind upon the main telescope. The ELT’s adaptive optics system
will provide an improvement of about a factor of 500 in the resolution,
compared to the best seeing conditions achieved so far without adaptive
optics.
The AdOptica consortium, partnered with INAF
(Istituto Nazionale di Astrofisica) as subcontractors, are responsible
for the design and manufacture of the quaternary mirror, which is to be
shipped to Chile by the end of 2022. Safran Reosc will manufacture the mirror shells, and also polish them.
ELT dome and structure
Dome construction
ELT concept.
The ELT dome will have a height of nearly 74 metres from the ground
and a diameter of 86 metres, making it the largest dome ever built for a
telescope. The dome will have a total mass of around 5000 tonnes, and
the telescope mounting and tube structure will have a total moving mass
of more than 3000 tonnes.
For the observing slit, two main designs were under study: one
with two sets of nested doors, and the current baseline design, i.e. a
single pair of large sliding doors. This pair of doors has a total width
of 45.3 m.
ESO signed a contract for its construction, together with the main structure of the telescopes, with the Italian ACe Consortium, consisting of Astaldi and Cimolai and the nominated subcontractor, Italy's EIE Group. The signature ceremony took place on 25 May 2016 at ESO’s Headquarters in Garching bei München, Germany.
The dome is to provide needed protection to the telescope in
inclement weather and during the day. A number of concepts for the dome
were evaluated. The baseline concept for the 40m-class ELT dome is a
nearly hemispherical dome, rotating atop a concrete pier, with curved
laterally opening doors. This is a re-optimisation from the previous
design, aimed at reducing the costs, and it is being revalidated to be
ready for construction.
One year after signing the contract, and after the laying of the
first stone ceremony in May 2017, the site was handed over to ACe,
signifying the beginning of the construction of the dome’s main
structure.
Astronomical performance
In terms of astronomical performance the dome is required to be able to track about the 1-degree zenithal
avoidance locus as well as preset to a new target within 5 minutes.
This requires the dome to be able to accelerate and move at angular
speeds of 2 degrees/sec (the linear speed is approximately 5 km/h).
The dome is designed to allow complete freedom to the telescope so
that it can position itself whether it is opened or closed. It will also
permit observations from the zenith down to 20 degrees from the
horizon.
Windscreen
With
such a large opening, the ELT dome requires the presence of a
windscreen to protect the telescope's mirrors (apart from the
secondary), from direct exposure to the wind. The baseline design of the
windscreen minimises the volume required to house it. Two spherical
blades, either side of the observing slit doors, slide in front of the
telescope aperture to restrict the wind.
Ventilation and air-conditioning
The
dome design ensures that the dome provides sufficient ventilation for
the telescope not to be limited by dome seeing. For this the dome is
also equipped with louvers, whereby the windscreen is designed to allow
them to fulfill their function.
Computational fluid dynamic simulations and wind tunnel work are
being carried out to study the airflow in and around the dome, as well
as the effectiveness of the dome and windscreen in protecting the
telescope.
Besides being designed for water-tightness, air-tightness is also
one of the requirements as it is critical to minimise the
air-conditioning load. The air-conditioning of the dome is necessary not
only to thermally prepare the telescope for the forthcoming night but
also in order to keep the telescope optics clean.
The air-conditioning of the telescope during the day is critical
and the current specifications permit the dome to cool the telescope and
internal volume by 10 °C over 12 hours.
Science goals
This is the official trailer for the ELT. The design for the ELT shown here is preliminary.
The ELT will search for extrasolar planets — planets orbiting other
stars. This will include not only the discovery of planets down to
Earth-like masses through indirect measurements of the wobbling motion
of stars perturbed by the planets that orbit them, but also the direct
imaging of larger planets and possibly even the characterisation of
their atmospheres. The telescope will attempt to image Earthlike exoplanets, which may be possible.
Furthermore, the ELT's suite of instruments will allow
astronomers to probe the earliest stages of the formation of planetary
systems and to detect water and organic molecules in protoplanetary
discs around stars in the making. Thus, the ELT will answer fundamental
questions regarding planet formation and evolution.
By probing the most distant objects the ELT will provide clues to
understanding the formation of the first objects that formed:
primordial stars, primordial galaxies and black holes and their
relationships. Studies of extreme objects like black holes will benefit
from the power of the ELT to gain more insight into time-dependent
phenomena linked with the various processes at play around compact
objects.
The ELT is designed to make detailed studies of the first
galaxies and to follow their evolution through cosmic time. Observations
of these early galaxies with the ELT will give clues that will help
understand how these objects form and evolve. In addition, the ELT will
be a unique tool for making an inventory of the changing content of the
various elements in the Universe with time, and to understand star
formation history in galaxies.
One of the goals of the ELT is the possibility of making a direct
measurement of the acceleration of the Universe's expansion. Such a
measurement would have a major impact on our understanding of the
Universe. The ELT will also search for possible variations in the
fundamental physical constants with time. An unambiguous detection of
such variations would have far-reaching consequences for our
comprehension of the general laws of physics.
Instrumentation
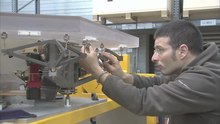
Play media
This
video shows engineers adjusting the complex support mechanisms that
control the shape and positioning of two of the 798 segments that will
form the complete primary mirror of the telescope.
The First ELT Instruments.
The telescope will have several science instruments. It will be
possible to switch from one instrument to another within minutes. The
telescope and dome will also be able to change positions on the sky and
start a new observation in a very short time.
Eight different instrument concepts and two post-focal adaptive
modules are currently being studied, and three have been selected for first light: MICADO, HARMONI and METIS. The others planning to become available at various points over the following decade. The instruments being designed or studied are:
- CODEX: a narrow-field, R=135 000 optical spectrograph
- EAGLE: a wide-field, multi-channel integral-field near-infrared (NIR) spectrograph, with multi-object adaptive optics
- EPICS: an optical/NIR planet imager and spectrograph with extreme adaptive optics
- HARMONI: a single field, wide-band integral field spectrograph
- METIS: a mid-infrared imager and spectrograph
- MICADO: a diffraction-limited near-infrared camera and spectrograph
- OPTIMOS: a wide-field visual multi-object spectrograph
- SIMPLE: a high-spectral-resolution NIR spectrograph
The two post-focal adaptive optics modules currently being studied are:
- ATLAS: a laser tomography adaptive optics module
- MAORY: a multi-conjugate adaptive optics module
Comparison
Comparison of nominal sizes of apertures of the Extremely Large Telescope and some notable optical telescopes
One of the largest optical telescope operating today is the Gran Telescopio Canarias, with a 10.4 m aperture and a light-collecting area of 74 m2. Other planned extremely large telescopes include the 25 m/368 m2 Giant Magellan Telescope and 30 m/655 m2 Thirty Meter Telescope,
which are also targeting the beginning of the 2020 decade for
completion. These other two telescopes roughly belong to the same next
generation of optical ground-based telescopes. Each design is much larger than previous telescopes. Even with the descale to 39.3 m the ELT is significantly larger than both other planned extremely large telescopes. It has the aim of observing the Universe in greater detail than the Hubble Space Telescope
by taking images 15 times sharper, although it is designed to be
complementary to space telescopes, which typically have very limited
observing time available. The ELT's 4.2-metre secondary mirror is the same size as the primary mirror on the William Herschel Telescope, the second largest optical telescope in Europe.
Name | Aperture diameter (m) |
Collecting area (m²) |
First light |
---|---|---|---|
Extremely Large Telescope (ELT) | 39.3 | 978 | 2024 |
Thirty Meter Telescope (TMT) | 30 | 655 | 2027 |
Giant Magellan Telescope (GMT) | 24.5 | 368 | 2024 |
Southern African Large Telescope (SALT) | 11.1 × 9.8 | 79 | 2005 |
Keck Telescopes | 10.0 | 76 | 1990, 1996 |
Gran Telescopio Canarias (GTC) | 10.4 | 74 | 2007 |
Very Large Telescope (VLT) | 8.2 | 50 (×4) | 1998–2000 |
Notes: Future first-light dates are provisional and likely to change. |
The ELT under ideal conditions has an angular resolution of 0.005 arcsecond which corresponds to separating two light sources 1 AU apart from 200 pc distance. At 0.03 arcseconds, the contrast is expected to be 108, sufficient to search for exoplanets. The unaided human eye has an angular resolution of 1 arcminute which corresponds to separating two light sources 30 cm apart from 1 km distance.