From Wikipedia, the free encyclopedia
Circulatory system | |
---|---|
![]()
The human circulatory system (simplified). Red indicates oxygenated blood, blue indicates deoxygenated.
(Not depicted are the intricate network of capillaries, as well as the entire lymphatic system.) |
|
Details | |
Latin | systema cardiovasculare |
Identifiers | |
TA | A12.0.00.000 |
FMA | 7161 |
Anatomical terminology |
The circulatory system, also called the cardiovascular system, is an organ system that permits
blood to circulate and transport nutrients (such as amino acids and electrolytes), oxygen, carbon dioxide, hormones, and blood cells to and from cells in the body to nourish it and help to fight diseases, stabilize body temperature and pH, and to maintain homeostasis.
The circulatory system is often seen to be composed of both the cardiovascular system, which distributes blood, and the lymphatic system, which circulates lymph.[1] These are two separate systems. The passage of lymph for example takes a lot longer than that of blood.[2] Blood is a fluid consisting of plasma, red blood cells, white blood cells, and platelets that is circulated by the heart through the vertebrate vascular system, carrying oxygen and nutrients to and waste materials away from all body tissues. Lymph is essentially recycled excess blood plasma after it has been filtered from the interstitial fluid (between cells) and returned to the lymphatic system. The cardiovascular (from Latin words meaning 'heart'-'vessel') system comprises the blood, heart, and blood vessels.[3] The lymph, lymph nodes, and lymph vessels form the lymphatic system, which returns filtered blood plasma from the interstitial fluid (between cells) as lymph.
While humans, as well as other vertebrates, have a closed cardiovascular system (meaning that the blood never leaves the network of arteries, veins and capillaries), some invertebrate groups have an open cardiovascular system. The lymphatic system, on the other hand, is an open system providing an accessory route for excess interstitial fluid to get returned to the blood.[4] The more primitive, diploblastic animal phyla lack circulatory systems.
Structure
Cardiovascular system
The essential components of the human cardiovascular system are the heart, blood, and blood vessels.[5] It includes: the pulmonary circulation, a "loop" through the lungs where blood is oxygenated; and the systemic circulation, a "loop" through the rest of the body to provide oxygenated blood. An average adult contains five to six quarts (roughly 4.7 to 5.7 liters) of blood, accounting for approximately 7% of their total body weight.[6] Blood consists of plasma, red blood cells, white blood cells, and platelets. Also, the digestive system works with the circulatory system to provide the nutrients the system needs to keep the heart pumping.[7]
The cardiovascular systems of humans are closed, meaning that the blood never leaves the network of blood vessels. In contrast, oxygen and nutrients diffuse across the blood vessel layers and enter interstitial fluid, which carries oxygen and nutrients to the target cells, and carbon dioxide and wastes in the opposite direction. The other component of the circulatory system, the lymphatic system, is not closed.
Heart
Coronary circulation
Coronary circulatory system provides a blood supply to the myocardium (the heart muscle). It arises from the aorta by two coronary arteries, the left and the right, and after nourishing the myocardium blood returns through the coronary veins into the coronary sinus and from this one into the right atrium. Back flow of blood through its opening during atrial systole is prevented by the Thebesian valve. The smallest cardiac veins drain directly into the heart chambers.[7]Pulmonary circulation

The pulmonary circulation as it passes from the heart. Showing both pulmonary trunk and bronchial arteries.
Oxygen deprived blood from the superior and inferior vena cava, enters the right atrium of the heart and flows through the tricuspid valve (right atrioventricular valve) into the right ventricle, from which it is then pumped through the pulmonary semilunar valve into the pulmonary artery to the lungs. Gas exchange occurs in the lungs, whereby CO
2 is released from the blood, and oxygen is absorbed. The pulmonary vein returns the now oxygen-rich blood to the left atrium.[7]
Systemic circulation
Systemic circulation is the circulation of the blood to all parts of the body except the lungs. Systemic circulation is the portion of the cardiovascular system which transports oxygenated blood away from the heart through the aorta from the left ventricle where the blood has been previously deposited from pulmonary circulation, to the rest of the body, and returns oxygen-depleted blood back to the heart. Systemic circulation is, distance-wise, much longer than pulmonary circulation, transporting blood to every part of the body.[7]Oxygen transportation
Development

The development of the circulatory system initially occurs by the process of vasculogenesis. The human arterial and venous systems develop from different embryonic areas. While the arterial system develops mainly from the aortic arches, the venous system arises from three bilateral veins during weeks 4 – 8 of human development. Fetal circulation does not include the use of the lungs.
Arterial development
The human arterial system originates from the aortic arches and from the dorsal aortae starting from week 4 of human development. Aortic arch 1 almost completely regresses except to form the maxillary arteries. Aortic arch 2 also completely regresses except to form the stapedial arteries. The definitive formation of the arterial system arise from aortic arches 3, 4 and 6, while aortic arch 5 completely regresses.The dorsal aortae are initially bilateral and then fuse to form the definitive dorsal aorta. Approximately 30 posterolateral branches arise off the aorta and will form the intercostal arteries, upper and lower extremity arteries, lumbar arteries and the lateral sacral arteries. The lateral branches of the aorta form the definitive renal, suprarrenal and gonadal arteries. Finally, the ventral branches of the aorta consist of the vitelline arteries and umbilical arteries. The vitelline arteries form the celiac, superior and inferior mesenteric arteries of the gastrointestinal tract. After birth, the umbilical arteries will form the internal iliac arteries.
Venous development
The human venous system develops mainly from the vitelline veins, the umbilical veins and the cardinal veins, all of which empty into the sinus venosus.Clinical significance
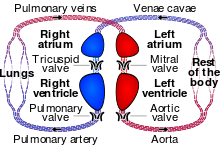
Measurement techniques
- Electrocardiogram—for cardiac electrophysiology
- Sphygmomanometer and stethoscope—for blood pressure
- Pulse meter—for cardiac function (heart rate, rhythm, dropped beats)
- Pulse—commonly used to determine the heart rate in absence of certain cardiac pathologies
- Heart rate variability—used to measure variations of time intervals between heart beats
- Nail bed blanching test—test for perfusion
- Vessel cannula or catheter pressure measurement—pulmonary wedge pressure or in older animal experiments.
Other animals
Other vertebrates
The circulatory systems of all vertebrates, as well as of annelids (for example, earthworms) and cephalopods (squids, octopuses and relatives) are closed, just as in humans. Still, the systems of fish, amphibians, reptiles, and birds show various stages of the evolution of the circulatory system.
In fish, the system has only one circuit, with the blood being pumped through the capillaries of the gills and on to the capillaries of the body tissues. This is known as single cycle circulation. The heart of fish is, therefore, only a single pump (consisting of two chambers).
In amphibians and most reptiles, a double circulatory system is used, but the heart is not always completely separated into two pumps. Amphibians have a three-chambered heart.
In reptiles, the ventricular septum of the heart is incomplete and the pulmonary artery is equipped with a sphincter muscle. This allows a second possible route of blood flow. Instead of blood flowing through the pulmonary artery to the lungs, the sphincter may be contracted to divert this blood flow through the incomplete ventricular septum into the left ventricle and out through the aorta. This means the blood flows from the capillaries to the heart and back to the capillaries instead of to the lungs. This process is useful to ectothermic (cold-blooded) animals in the regulation of their body temperature.
Birds and mammals show complete separation of the heart into two pumps, for a total of four heart chambers; it is thought[citation needed] that the four-chambered heart of birds evolved independently from that of mammals.
Open circulatory system
The open circulatory system is a system in which a fluid in a cavity called the hemocoel bathes the organs directly with oxygen and nutrients and there is no distinction between blood and interstitial fluid; this combined fluid is called hemolymph or haemolymph.[8] Muscular movements by the animal during locomotion can facilitate hemolymph movement, but diverting flow from one area to another is limited. When the heart relaxes, blood is drawn back toward the heart through open-ended pores (ostia).Hemolymph fills all of the interior hemocoel of the body and surrounds all cells. Hemolymph is composed of water, inorganic salts (mostly Na+, Cl−, K+, Mg2+, and Ca2+), and organic compounds (mostly carbohydrates, proteins, and lipids). The primary oxygen transporter molecule is hemocyanin.
There are free-floating cells, the hemocytes, within the hemolymph. They play a role in the arthropod immune system.
Absence of circulatory system
Circulatory systems are absent in some animals, including flatworms (phylum Platyhelminthes).Their body cavity has no lining or enclosed fluid. Instead a muscular pharynx leads to an extensively branched digestive system that facilitates direct diffusion of nutrients to all cells. The flatworm's dorso-ventrally flattened body shape also restricts the distance of any cell from the digestive system or the exterior of the organism. Oxygen can diffuse from the surrounding water into the cells, and carbon dioxide can diffuse out. Consequently every cell is able to obtain nutrients, water and oxygen without the need of a transport system.
Some animals, such as jellyfish, have more extensive branching from their gastrovascular cavity (which functions as both a place of digestion and a form of circulation), this branching allows for bodily fluids to reach the outer layers, since the digestion begins in the inner layers.
History of discovery
Human anatomical chart of blood vessels, with heart, lungs, liver and kidneys included. Other organs are numbered and arranged around it. Before cutting out the figures on this page, Vesalius suggests that readers glue the page onto parchment and gives instructions on how to assemble the pieces and paste the multilayered figure onto a base "muscle man" illustration. "Epitome", fol.14a. HMD Collection, WZ 240 V575dhZ 1543.
The earliest known writings on the circulatory system are found in the Ebers Papyrus (16th century BCE), an ancient Egyptian medical papyrus containing over 700 prescriptions and remedies, both physical and spiritual. In the papyrus, it acknowledges the connection of the heart to the arteries. The Egyptians thought air came in through the mouth and into the lungs and heart. From the heart, the air travelled to every member through the arteries. Although this concept of the circulatory system is only partially correct, it represents one of the earliest accounts of scientific thought.
In the 6th century BCE, the knowledge of circulation of vital fluids through the body was known to the Ayurvedic physician Sushruta in ancient India.[9] He also seems to have possessed knowledge of the arteries, described as 'channels' by Dwivedi & Dwivedi (2007).[9] The valves of the heart were discovered by a physician of the Hippocratean school around the 4th century BCE. However their function was not properly understood then. Because blood pools in the veins after death, arteries look empty. Ancient anatomists assumed they were filled with air and that they were for transport of air.
The Greek physician, Herophilus, distinguished veins from arteries but thought that the pulse was a property of arteries themselves. Greek anatomist Erasistratus observed that arteries that were cut during life bleed. He ascribed the fact to the phenomenon that air escaping from an artery is replaced with blood that entered by very small vessels between veins and arteries. Thus he apparently postulated capillaries but with reversed flow of blood.[10]
In 2nd century AD Rome, the Greek physician Galen knew that blood vessels carried blood and identified venous (dark red) and arterial (brighter and thinner) blood, each with distinct and separate functions. Growth and energy were derived from venous blood created in the liver from chyle, while arterial blood gave vitality by containing pneuma (air) and originated in the heart. Blood flowed from both creating organs to all parts of the body where it was consumed and there was no return of blood to the heart or liver. The heart did not pump blood around, the heart's motion sucked blood in during diastole and the blood moved by the pulsation of the arteries themselves.
Galen believed that the arterial blood was created by venous blood passing from the left ventricle to the right by passing through 'pores' in the interventricular septum, air passed from the lungs via the pulmonary artery to the left side of the heart. As the arterial blood was created 'sooty' vapors were created and passed to the lungs also via the pulmonary artery to be exhaled.
In 1025, The Canon of Medicine by the Persian physician, Avicenna, "erroneously accepted the Greek notion regarding the existence of a hole in the ventricular septum by which the blood traveled between the ventricles." Despite this, Avicenna "correctly wrote on the cardiac cycles and valvular function", and "had a vision of blood circulation" in his Treatise on Pulse.[11][verification needed] While also refining Galen's erroneous theory of the pulse, Avicenna provided the first correct explanation of pulsation: "Every beat of the pulse comprises two movements and two pauses. Thus, expansion : pause : contraction : pause. [...] The pulse is a movement in the heart and arteries ... which takes the form of alternate expansion and contraction."[12]
In 1242, the Arabian physician, Ibn al-Nafis, became the first person to accurately describe the process of pulmonary circulation, for which he is sometimes considered the father of circulatory physiology.[13][not in citation given] Ibn al-Nafis stated in his Commentary on Anatomy in Avicenna's Canon:
"...the blood from the right chamber of the heart must arrive at the left chamber but there is no direct pathway between them. The thick septum of the heart is not perforated and does not have visible pores as some people thought or invisible pores as Galen thought. The blood from the right chamber must flow through the vena arteriosa (pulmonary artery) to the lungs, spread through its substances, be mingled there with air, pass through the arteria venosa (pulmonary vein) to reach the left chamber of the heart and there form the vital spirit..."In addition, Ibn al-Nafis had an insight into what would become a larger theory of the capillary circulation. He stated that "there must be small communications or pores (manafidh in Arabic) between the pulmonary artery and vein," a prediction that preceded the discovery of the capillary system by more than 400 years.[14] Ibn al-Nafis' theory, however, was confined to blood transit in the lungs and did not extend to the entire body.
Michael Servetus was the first European to describe the function of pulmonary circulation, although his achievement was not widely recognized at the time, for a few reasons. He firstly described it in the "Manuscript of Paris"[15][16] (near 1546), but this work was never published. And later he published this description, but in a theological treatise, Christianismi Restitutio, not in a book on medicine. Only three copies of the book survived, the rest were burned shortly after its publication in 1553 because of persecution of Servetus by religious authorities. Better known was its discovery by Vesalius's successor at Padua, Realdo Colombo, in 1559.

Image of veins from William Harvey's Exercitatio Anatomica de Motu Cordis et Sanguinis in Animalibus
Finally, William Harvey, a pupil of Hieronymus Fabricius (who had earlier described the valves of the veins without recognizing their function), performed a sequence of experiments, and published Exercitatio Anatomica de Motu Cordis et Sanguinis in Animalibus in 1628, which "demonstrated that there had to be a direct connection between the venous and arterial systems throughout the body, and not just the lungs. Most importantly, he argued that the beat of the heart produced a continuous circulation of blood through minute connections at the extremities of the body. This is a conceptual leap that was quite different from Ibn al-Nafis' refinement of the anatomy and bloodflow in the heart and lungs."[17] This work, with its essentially correct exposition, slowly convinced the medical world. However, Harvey was not able to identify the capillary system connecting arteries and veins; these were later discovered by Marcello Malpighi in 1661.
In 1956, André Frédéric Cournand, Werner Forssmann and Dickinson W. Richards were awarded the Nobel Prize in Medicine "for their discoveries concerning heart catheterization and pathological changes in the circulatory system."[18]