From Wikipedia, the free encyclopedia
At
the neuromuscular junction, the nerve fiber is able to transmit a
signal to the muscle fiber by releasing ACh (and other substances),
causing muscle contraction.
Muscles
will contract or relax when they receive signals from the nervous
system. The neuromuscular junction is the site of the signal exchange.
The steps of this process in vertebrates occur as follows:(1) The action
potential reaches the axon terminal. (2) Voltage-dependent calcium
gates open, allowing calcium to enter the axon terminal. (3)
Neurotransmitter vesicles fuse with the presynaptic membrane and ACh is
released into the synaptic cleft via exocytosis. (4) ACh binds to
postsynaptic receptors on the sarcolemma. (5) This binding causes ion
channels to open and allows sodium and other cations to flow across the
membrane into the muscle cell. (6) The flow of sodium ions across the
membrane into and potassium ions out of the muscle cell generates an
action potential which travels to the myofibril and results in muscle
contraction.Labels:A: Motor Neuron AxonB: Axon TerminalC. Synaptic
CleftD. Muscle CellE. Part of a Myofibril
Neuromuscular junctions |
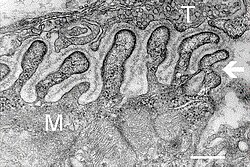
Electron micrograph
showing a cross section through the neuromuscular junction. T is the
axon terminal, M is the muscle fiber. The arrow shows junctional folds
with basal lamina. Active zones are visible on the tips between the folds. Scale is 0.3 µm. Source: NIMH
|

Detailed view of a neuromuscular junction:
|
Details |
Identifiers |
Latin | synapssis neuromuscularis; junctio neuromuscularis |
MeSH | D009469 |
TH | H2.00.06.1.02001 |
FMA | 61803 |
Neuromuscular junction diseases can be of
genetic and
autoimmune origin. Genetic disorders, such as
Duchenne muscular dystrophy, can arise from mutated structural proteins that comprise the neuromuscular junction, whereas autoimmune diseases, such as
myasthenia gravis, occur when antibodies are produced against nicotinic acetylcholine receptors on the sarcolemma.
Structure and function
Quantal transmission
At the
neuromuscular junction presynaptic motor axons terminate 30 nanometers from the cell membrane or
sarcolemma of a muscle fiber. The sarcolemma at the junction has
invaginations called postjunctional folds, which increase its surface area facing the synaptic cleft. These postjunctional folds form the motor endplate, which is studded with
nicotinic acetylcholine receptors (nAChRs) at a density of 10,000 receptors/micrometer
2.
The presynaptic axons terminate in bulges called terminal boutons (or
presynaptic terminals) that project toward the postjunctional folds of
the sarcolemma. In the frog each motor nerve terminal contains about
300,000
vesicles,
with an average diameter of 0.05 micrometers. The vesicles contain
acetylcholine. Some of these vesicles are gathered into groups of
fifty, positioned at active zones close to the nerve membrane. Active
zones are about 1 micrometer apart.
The 30 nanometer cleft between nerve ending and endplate contains a
meshwork of acetylcholinesterase (AChE) at a density of 2,600 enzyme
molecules/micrometer
2, held in place by the structural proteins
dystrophin and
rapsyn. Also present is the
receptor tyrosine kinase protein
MuSK, a signaling protein involved in the development of the neuromuscular junction, which is also held in place by rapsyn.
About once every second in a resting junction randomly one of the synaptic vesicles fuses with the presynaptic neuron's
cell membrane in a process mediated by
SNARE proteins. Fusion results in the emptying of the vesicle's contents of 7000-10,000 acetylcholine molecules into the
synaptic cleft, a process known as
exocytosis.
Consequently exocytosis releases acetylcholine in packets that are
called quanta. The acetylcholine quantum diffuses through the
acetylcholinesterase meshwork, where the high local transmitter
concentration occupies all of the binding sites on the enzyme in its
path. The acetylcholine that reaches the endplate activates ~2,000
acetylcholine receptors, opening their ion channels which permits sodium
ions to move into the endplate producing a depolarization of ~0.5 mV
known as a miniature endplate potential (MEPP). By the time the
acetylcholine is released from the receptors the acetylcholinesterase
has destroyed its bound ACh, which takes about ~0.16 ms, and hence is
available to destroy the ACh released from the receptors.
When the motor nerve is stimulated there is a delay of only 0.5
to 0.8 msec between the arrival of the nerve impulse in the motor nerve
terminals and the first response of the endplate The arrival of the motor nerve
action potential at the presynaptic neuron terminal opens
voltage-dependent calcium channels and Ca
2+ ions flow from the extracellular fluid into the presynaptic neuron's
cytosol. This influx of Ca
2+ causes several hundred
neurotransmitter-containing
vesicles to fuse with the presynaptic neuron's cell membrane through
SNARE
proteins to release their acetylcholine quanta by exocytosis. The
endplate depolarization by the released acetylcholine is called an
endplate potential (EPP). The EPP is accomplished when ACh binds the
nicotinic acetylcholine receptors (nAChR) at the motor end plate, and
causes an influx of sodium ions. This influx of sodium ions generates
the EPP (depolarization), and triggers an action potential which travels
along the sarcolemma and into the muscle fiber via the transverse
tubules (T-tubules) by means of voltage-gated sodium channels. The conduction of action potentials along the transverse tubules stimulates the opening of voltage-gated Ca
2+ channels which are mechanically coupled to Ca
2+ release channels in the sarcoplasmic reticulum. The Ca
2+
then diffuses out of the sarcoplasmic reticulum to the myofibrils so it
can stimulate contraction. The endplate potential is thus responsible
for setting up an action potential in the muscle fiber which triggers
muscle contraction. The transmission from nerve to muscle is so rapid
because each quantum of acetylcholine reaches the endplate in
millimolar concentrations, high enough to combine with a receptor with a
low affinity, which then swiftly releases the bound transmitter.
Acetylcholine receptors
When ligands bind to the receptor, the ion channel portion of the receptor opens, allowing ions to pass across the cell membrane.
AChRs at the skeletal neuromuscular junction form heteropentamers composed of two α, one β, one ɛ, and one δ subunits. When a single ACh ligand binds to one of the α subunits of the ACh receptor it induces a
conformational change at the interface with the second AChR α subunit. This conformational change results in the increased
affinity of the second α subunit for a second ACh ligand. AChRs therefore exhibit a sigmoidal dissociation curve due to this
cooperative binding.
The presence of the inactive, intermediate receptor structure with a
single-bound ligand keeps ACh in the synapse that might otherwise be
lost by
cholinesterase hydrolysis or diffusion. The persistence of these ACh ligands in the synapse can cause a prolonged post-synaptic response.
Development
The
development of the neuromuscular junction requires signaling from both
the motor neuron's terminal and the muscle cell's central region. During
development, muscle cells produce acetylcholine receptors (AChRs) and
express them in the central regions in a process called prepatterning.
Agrin, a heparin
proteoglycan,
and MuSK kinase are thought to help stabilize the accumulation of AChR
in the central regions of the myocyte. MuSK is a receptor
tyrosine kinase—meaning that it induces cellular signaling by binding
phosphate molecules to self regions like
tyrosines, and to other targets in the
cytoplasm. Upon activation by its ligand agrin, MuSK signals via two proteins called "
Dok-7" and "
rapsyn", to induce "clustering" of acetylcholine receptors.
ACh release by developing motor neurons produces postsynaptic
potentials in the muscle cell that positively reinforces the
localization and stabilization of the developing neuromuscular junction.
These findings were demonstrated in part by mouse "
knockout"
studies. In mice which are deficient for either agrin or MuSK, the
neuromuscular junction does not form. Further, mice deficient in
Dok-7 did not form either acetylcholine receptor clusters or neuromuscular synapses.
The development of neuromuscular junctions is mostly studied in
model organisms, such as rodents. In addition, in 2015 an all-human
neuromuscular junction has been created in vitro using human
embryonic stem cells and somatic muscle stem cells. In this model presynaptic
motor neurons are activated by
optogenetics and in response synaptically connected muscle fibers twitch upon light stimulation.
Research methods
José del Castillo and Bernard Katz used ionophoresis to determine the location and density of
nicotinic acetylcholine receptors
(nAChRs) at the neuromuscular junction. With this technique, a
microelectrode was placed inside the motor endplate of the muscle fiber,
and a micropipette filled with acetylcholine (ACh) is placed directly
in front of the endplate in the synaptic cleft. A positive voltage was
applied to the tip of the micropipette, which caused a burst of
positively charged ACh molecules to be released from the pipette. These
ligands flowed into the space representing the synaptic cleft and bound
to AChRs. The intracellular microelectrode monitored the
amplitude of the
depolarization
of the motor endplate in response to ACh binding to nicotinic
(ionotropic) receptors. Katz and del Castillo showed that the amplitude
of the depolarization (
excitatory postsynaptic potential)
depended on the proximity of the micropipette releasing the ACh ions to
the endplate. The farther the micropipette was from the motor
endplate, the smaller the depolarization was in the muscle fiber. This
allowed the researchers to determine that the nicotinic receptors were
localized to the motor endplate in high density.
Toxins are also used to determine the location of acetylcholine receptors at the neuromuscular junction.
α-Bungarotoxin is a toxin found in the snake species
Bungarus multicinctus that acts as an ACh antagonist and binds to AChRs irreversibly. By coupling assayable enzymes such as
horseradish peroxidase (HRP) or fluorescent proteins such as
green fluorescent protein (GFP) to the α-bungarotoxin, AChRs can be visualized and quantified.
Toxins that affect the neuromuscular junction
Nerve gases
Botulinum toxin
Botulinum toxin
(aka botulinum neurotoxin, BoNT, and sold under the trade name Botox)
inhibits the release of acetylcholine at the neuromuscular junction by
interfering with SNARE proteins. This toxin crosses into the nerve terminal through the process of
endocytosis and subsequently interferes with SNARE proteins, which are necessary for ACh release. By doing so, it induces a transient
flaccid paralysis
and chemical denervation localized to the striated muscle that it has
affected. The inhibition of the ACh release does not set in until
approximately two weeks after the injection is made. Three months after
the inhibition occurs, neuronal activity begins to regain partial
function, and six months, complete neuronal function is regained.
Tetanus toxin
Tetanus toxin, also known as
tetanospasmin is a potent neurotoxin produced by
Clostridium tetani and causes the disease state, tetanus. The LD
50
of this toxin has been measured to be approximately 1 ng/kg, making it
second only to Botulinum toxin D as the deadliest toxin in the world. It
functions very similarly to botunlinum neurotoxin (BoNT) by attaching
and endocytosing into the presynaptic nerve terminal and interfering
with SNARE protein complexes. It differs from BoNT in a few ways, most
apparently in its end state, wherein tetanospasmin demonstrates a rigid /
spastic paralysis as opposed to the flaccid paralysis demonstrated with BoNT.
Latrotoxin
Latrotoxin
(α-Latrotoxin) found in venom of widow spiders also affects the
neuromuscular junction by causing the release of acetylcholine from the
presynaptic cell. Mechanisms of action include binding to receptors on
the presynaptic cell activating the
IP3/DAG pathway
and release of calcium from intracellular stores and pore formation
resulting in influx of calcium ions directly. Either mechanism causes
increased calcium in presynaptic cell, which then leads to release of
synaptic vesicles of acetylcholine. Latrotoxin causes pain, muscle
contraction and if untreated potentially paralysis and death.
Snake venom
Snake venoms act as toxins at the neuromuscular junction and can induce weakness and
paralysis. Venoms can act as both presynaptic and postsynaptic neurotoxins.
Presynaptic neurotoxins, commonly known as β-neurotoxins, affect
the presynaptic regions of the neuromuscular junction. The majority of
these neurotoxins act by inhibiting the release of neurotransmitters,
such as acetylcholine, into the synapse between neurons. However, some
of these toxins have also been known to enhance neurotransmitter
release. Those that inhibit neurotransmitter release create a
neuromuscular blockade
that prevents signaling molecules from reaching their postsynaptic
target receptors. In doing so, the victim of these snake bite suffer
from profound weakness. Such neurotoxins do not respond well to
anti-venoms. After one hour of inoculation of these toxins, including
notexin and
taipoxin, many of the affected nerve terminals show signs of irreversible physical damage, leaving them devoid of any
synaptic vesicles.
Postsynaptic neurotoxins, otherwise known as α-neurotoxins, act
oppositely to the presynaptic neurotoxins by binding to the postsynaptic
acetylcholine receptors. This prevents interaction between the
acetylcholine released by the presynaptic terminal and the receptors on
the postsynaptic cell. In effect, the opening of sodium channels
associated with these acetylcholine receptors is prohibited, resulting
in a neuromuscular blockade, similar to the effects seen due to
presynaptic neurotoxins. This causes paralysis in the muscles involved
in the affected junctions. Unlike presynaptic neurotoxins, postsynaptic
toxins are more easily affected by anti-venoms, which accelerate the
dissociation of the toxin from the receptors, ultimately causing a
reversal of paralysis. These neurotoxins experimentally and
qualitatively aid in the study of acetylcholine receptor
density and
turnover, as well as in studies observing the direction of
antibodies toward the affected acetylcholine receptors in patients diagnosed with
myasthenia gravis.
Diseases
Any
disorder
that compromises the synaptic transmission between a motor neuron and a
muscle cell is categorized under the umbrella term of neuromuscular
diseases. These disorders can be
inherited
or acquired and can vary in their severity and mortality. In general,
most of these disorders tend to be caused by mutations or autoimmune
disorders. Autoimmune disorders, in the case of neuromuscular diseases,
tend to be
humoral mediated,
B cell mediated, and result in an
antibody improperly created against a motor neuron or muscle fiber protein that interferes with synaptic transmission or signaling.
Autoimmune
Myasthenia gravis
Myasthenia gravis
is an autoimmune disorder where the body makes antibodies against
either the acetylcholine receptor (AchR) (in 80% of cases), or against
postsynaptic muscle-specific kinase (MuSK) (0–10% of cases). In
seronegative myasthenia gravis
low density lipoprotein receptor-related protein 4 is targeted by
IgG1,
which acts as a competitive inhibitor of its ligand, preventing the
ligand from binding its receptor. It is not known if seronegative
myasthenia gravis will respond to standard therapies.
Neonatal MG
Neonatal
MG is an autoimmune disorder that affects 1 in 8 children born to
mothers who have been diagnosed with myasthenia gravis (MG). MG can be
transferred from the mother to the fetus by the movement of AChR
antibodies through the
placenta.
Signs of this disease at birth include weakness, which responds to
anticholinesterase medications, as well as fetal akinesia, or the lack
of fetal movement. This form of the disease is transient, lasting for
about three months. However, in some cases, neonatal MG can lead to
other health effects, such as arthrogryposis and even fetal death. These
conditions are thought to be initiated when maternal AChR antibodies
are directed to the
fetal AChR and can last until the 33rd week of
gestation, when the γ subunit of AChR is replaced by the ε subunit.
Lambert-Eaton myasthenic syndrome
Lambert-Eaton myasthenic syndrome
(LEMS) is an autoimmune disorder that affects the presynaptic portion
of the neuromuscular junction. This rare disease can be marked by a
unique triad of symptoms: proximal muscle weakness,
autonomic dysfunction, and areflexia. Proximal muscle weakness is a product of
pathogenic
autoantibodies directed against P/Q-type voltage-gated calcium
channels, which in turn leads to a reduction of acetylcholine release
from motor nerve terminals on the presynaptic cell. Examples of
autonomic dysfunction caused by LEMS include
erectile dysfunction in men,
constipation, and, most commonly,
dry mouth. Less common dysfunctions include
dry eyes and altered
perspiration.
Areflexia is a condition in which tendon reflexes are reduced and it may subside temporarily after a period of exercise.
Treatment for LEMS consists of using 3,4-diaminopyridine as a
first measure, which serves to increase the compound muscle action
potential as well as muscle strength by lengthening the time that
voltage-gated calcium channels remain open after blocking voltage-gated
potassium channels. In the US, treatment with 3,4-diaminopyridine for
eligible LEMS patients is available at no cost under an expanded access
program. Further treatment includes the use of
prednisone and
azathioprine in the event that 3,4-diaminopyridine does not aid in treatment.
Neuromyotonia
Neuromyotonia
(NMT), otherwise known as Isaac’s syndrome, is unlike many other
diseases present at the neuromuscular junction. Rather than causing
muscle weakness, NMT leads to the hyperexcitation of motor nerves. NMT
causes this hyperexcitation by producing longer depolarizations by
down-regulating
voltage-gated potassium channels,
which causes greater neurotransmitter release and repetitive firing.
This increase in rate of firing leads to more active transmission and as
a result, greater muscular activity in the affected individual. NMT is
also believed to be of
autoimmune origin due to its associations with autoimmune symptoms in the individual affected.
Genetic
Congenital myasthenic syndromes
Congenital myasthenic syndromes
(CMS) are very similar to both MG and LEMS in their functions, but the
primary difference between CMS and those diseases is that CMS is of
genetic origins. Specifically, these syndromes are diseases incurred due
to mutations, typically
recessive,
in 1 of at least 10 genes that affect presynaptic, synaptic, and
postsynaptic proteins in the neuromuscular junction. Such mutations
usually arise in the ε-subunit of AChR,
thereby affecting the kinetics and expression of the receptor itself.
Single nucleotide substitutions or deletions may cause loss of function
in the subunit. Other
mutations, such as those affecting
acetylcholinesterase and
acetyltransferase, can also cause the expression of CMS, with the latter being associated specifically with episodic
apnea.
These syndromes can present themselves at different times within the
life of an individual. They may arise during the fetal phase, causing
fetal
akinesia, or the perinatal period, during which certain conditions, such as
arthrogryposis,
ptosis,
hypotonia,
ophthalmoplegia,
and feeding or breathing difficulties, may be observed. They could also
activate during adolescence or adult years, causing the individual to
develop slow-channel syndrome.
Treatment for particular subtypes of CMS (postsynaptic fast-channel CMS) is similar to treatment for other neuromuscular disorders.
3,4-Diaminopyridine, the first-line treatment for LEMS, is under development as an orphan drug for CMS in the US, and available to eligible patients under an expanded access program at no cost.
Bulbospinal muscular atrophy
Bulbospinal muscular atrophy, also known as Kennedy’s disease, is a rare
recessive trinucleotide, polyglutamine disorder that is linked to the
X chromosome.
Because of its linkage to the X chromosome, it is typically transmitted
through females. However, Kennedy’s disease is only present in adult
males and the onset of the disease is typically later in life. This
disease is specifically caused by the expansion of a CAG-tandem repeat
in exon 1 found on the androgen-receptor (AR) gene on
chromosome Xq11-12.
Poly-Q-expanded AR accumulates in the nuclei of cells, where it begins
to fragment. After fragmentation, degradation of the cell begins,
leading to a loss of both motor neurons and
dorsal root ganglia.
Symptoms of Kennedy’s disease include weakness and wasting of the facial
bulbar and extremity muscles, as well as sensory and endocrinological disturbances, such as
gynecomastia and reduced
fertility. Other symptoms include elevated
testosterone and other sexual hormone levels, development of hyper-CK-emia, abnormal conduction through motor and sensory nerves, and
neuropathic or in rare cases
myopathic alterations on biopsies of muscle cells.
Duchenne muscular dystrophy
Duchenne muscular dystrophy is an X-linked genetic disorder that results in the absence of the structural protein
dystrophin
at the neuromuscular junction. It affects 1 in 3,600–6,000 males and
frequently causes death by the age of 30. The absence of dystrophin
causes muscle
degeneration, and patients present with the following symptoms: abnormal
gait,
hypertrophy in the calf muscles, and elevated
creatine kinase. If left untreated, patients may suffer from
respiratory distress, which can lead to death.