
Carbon sequestration is the process of storing carbon in a carbon pool. It plays a crucial role in limiting climate change by reducing the amount of carbon dioxide in the atmosphere. There are two main types of carbon sequestration: biologic (also called biosequestration) and geologic.
Biologic carbon sequestration is a naturally occurring process as part of the carbon cycle. Humans can enhance it through deliberate actions and use of technology. Carbon dioxide (CO
2) is naturally captured from the atmosphere
through biological, chemical, and physical processes. These processes
can be accelerated for example through changes in land use and
agricultural practices, called carbon farming. Artificial processes have also been devised to produce similar effects. This approach is called carbon capture and storage. It involves using technology to capture and sequester (store) CO
2 that is produced from human activities underground or under the sea bed.
Plants, such as forests and kelp beds, absorb carbon dioxide from the air as they grow, and bind it into biomass. However, these biological stores may be temporary carbon sinks, as long-term sequestration cannot be guaranteed. Wildfires, disease, economic pressures, and changing political priorities may release the sequestered carbon back into the atmosphere.
Carbon dioxide that has been removed from the atmosphere can also be stored in the Earth's crust by injecting it underground, or in the form of insoluble carbonate salts. The latter process is called mineral sequestration. These methods are considered non-volatile because they not only remove carbon dioxide from the atmosphere but also sequester it indefinitely. This means the carbon is "locked away" for thousands to millions of years.
To enhance carbon sequestration processes in oceans the following technologies have been proposed: seaweed farming, ocean fertilization, artificial upwelling, basalt storage, mineralization and deep sea sediments, and adding bases to neutralize acids. However, none have achieved large scale application so far.
Terminology
The term carbon sequestration is used in different ways in the literature and media. The IPCC Sixth Assessment Report defines it as "The process of storing carbon in a carbon pool". Subsequently, a pool is defined as "a reservoir in the Earth system where elements, such as carbon and nitrogen, reside in various chemical forms for a period of time".
The United States Geological Survey (USGS) defines carbon sequestration as follows: "Carbon sequestration is the process of capturing and storing atmospheric carbon dioxide." Therefore, the difference between carbon sequestration and carbon capture and storage (CCS) is sometimes blurred in the media. The IPCC, however, defines CCS as "a process in which a relatively pure stream of carbon dioxide (CO2) from industrial sources is separated, treated and transported to a long-term storage location".
Roles
In nature
Carbon sequestration is part of the natural carbon cycle by which carbon is exchanged among the biosphere, pedosphere (soil), geosphere, hydrosphere, and atmosphere of Earth. Carbon dioxide is naturally captured from the atmosphere through biological, chemical or physical processes, and stored in long-term reservoirs.
Plants, such as forests and kelp beds, absorb carbon dioxide from the air as they grow, and bind it into biomass. However, these biological stores are considered volatile carbon sinks as the long-term sequestration cannot be guaranteed. Events such as wildfires or disease, economic pressures and changing political priorities can result in the sequestered carbon being released back into the atmosphere.
In climate change mitigation
Carbon sequestration - when acting as a carbon sink -[clarification needed] helps to mitigate climate change and thus reduce harmful effects of climate change. It helps to slow the atmospheric and marine accumulation of greenhouse gases, which is mainly carbon dioxide released by burning fossil fuels.
Carbon sequestration, when applied for climate change mitigation, can either build on enhancing naturally occurring carbon sequestration or use technology for carbon sequestration processes.
Within the carbon capture and storage approaches, carbon sequestration refers to the storage component. Artificial carbon storage technologies can be applied, such as gaseous storage in deep geological formations (including saline formations and exhausted gas fields), and solid storage by reaction of CO2 with metal oxides to produce stable carbonates.
For carbon to be sequestered artificially (i.e. not using the natural processes of the carbon cycle) it must first be captured, or it must be significantly delayed or prevented from being re-released into the atmosphere (by combustion, decay, etc.) from an existing carbon-rich material, by being incorporated into an enduring usage (such as in construction). Thereafter it can be passively stored or remain productively utilized over time in a variety of ways. For instance, upon harvesting, wood (as a carbon-rich material) can be incorporated into construction or a range of other durable products, thus sequestering its carbon over years or even centuries.
Biological carbon sequestration on land
Biological carbon sequestration (also called biosequestration) is the capture and storage of the atmospheric greenhouse gas carbon dioxide by continual or enhanced biological processes. This form of carbon sequestration occurs through increased rates of photosynthesis via land-use practices such as reforestation and sustainable forest management. Land-use changes that enhance natural carbon capture have the potential to capture and store large amounts of carbon dioxide each year. These include the conservation, management, and restoration of ecosystems such as forests, peatlands, wetlands, and grasslands, in addition to carbon sequestration methods in agriculture. Methods and practices exist to enhance soil carbon sequestration in both agriculture and forestry.
Forestry

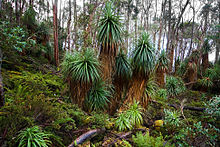

Forests are an important part of the global carbon cycle because trees and plants absorb carbon dioxide through photosynthesis. Therefore, they play an important role in climate change mitigation. By removing the greenhouse gas carbon dioxide from the air, forests function as terrestrial carbon sinks, meaning they store large amounts of carbon in the form of biomass, encompassing roots, stems, branches, and leaves. Throughout their lifespan, trees continue to sequester carbon, storing atmospheric CO2 long-term. Sustainable forest management, afforestation, reforestation are therefore important contributions to climate change mitigation.
An important consideration in such efforts is that forests can turn from sinks to carbon sources. In 2019 forests took up a third less carbon than they did in the 1990s, due to higher temperatures, droughts and deforestation. The typical tropical forest may become a carbon source by the 2060s.
Researchers have found that, in terms of environmental services, it is better to avoid deforestation than to allow for deforestation to subsequently reforest, as the former leads to irreversible effects in terms of biodiversity loss and soil degradation. Furthermore, the probability that legacy carbon will be released from soil is higher in younger boreal forest. Global greenhouse gas emissions caused by damage to tropical rainforests may have been substantially underestimated until around 2019. Additionally, the effects of af- or reforestation will be farther in the future than keeping existing forests intact. It takes much longer − several decades − for the benefits for global warming to manifest to the same carbon sequestration benefits from mature trees in tropical forests and hence from limiting deforestation. Therefore, scientists consider "the protection and recovery of carbon-rich and long-lived ecosystems, especially natural forests" to be "the major climate solution".
The planting of trees on marginal crop and pasture lands helps to incorporate carbon from atmospheric CO
2 into biomass.
For this carbon sequestration process to succeed the carbon must not
return to the atmosphere from biomass burning or rotting when the trees
die.
To this end, land allotted to the trees must not be converted to other
uses. Alternatively, the wood from them must itself be sequestered,
e.g., via biochar, bioenergy with carbon capture and storage, landfill or stored by use in construction.
Earth offers enough room to plant an additional 1.2 trillion trees. Planting and protecting them would offset some 10 years of CO2 emissions and sequester 205 billion tons of carbon. This approach is supported by the Trillion Tree Campaign. Restoring all degraded forests world-wide would capture about 205 billion tons of carbon in total, which is about two-thirds of all carbon emissions.
Life expectancy of forests varies throughout the world, influenced by tree species, site conditions and natural disturbance patterns. In some forests, carbon may be stored for centuries, while in other forests, carbon is released with frequent stand replacing fires. Forests that are harvested prior to stand replacing events allow for the retention of carbon in manufactured forest products such as lumber. However, only a portion of the carbon removed from logged forests ends up as durable goods and buildings. The remainder ends up as sawmill by-products such as pulp, paper and pallets. If all new construction globally utilized 90% wood products, largely via adoption of mass timber in low rise construction, this could sequester 700 million net tons of carbon per year. This is in addition to the elimination of carbon emissions from the displaced construction material such as steel or concrete, which are carbon-intense to produce.
A meta-analysis found that mixed species plantations would increase carbon storage alongside other benefits of diversifying planted forests.
Although a bamboo forest stores less total carbon than a mature forest of trees, a bamboo plantation sequesters carbon at a much faster rate than a mature forest or a tree plantation. Therefore, the farming of bamboo timber may have significant carbon sequestration potential.
The Food and Agriculure Organization (FAO) reported that: "The total carbon stock in forests decreased from 668 gigatonnes in 1990 to 662 gigatonnes in 2020". In Canada's boreal forests as much as 80% of the total carbon is stored in the soils as dead organic matter.
Carbon offset programs are planting millions of fast-growing trees per year to reforest tropical lands. Over their typical 40-year lifetime, one million of these trees can sequester up to one million tons of carbon dioxide.
The IPCC Sixth Assessment Report says: "Secondary forest regrowth and restoration of degraded forests and non-forest ecosystems can play a large role in carbon sequestration (high confidence) with high resilience to disturbances and additional benefits such as enhanced biodiversity."
At the beginning of the 21st century, interest in reforestation grew over its potential to mitigate climate change. Even without displacing agriculture and cities, earth can sustain almost one billion hectares of new forests. This would remove 25% of carbon dioxide from the atmosphere and reduce its concentration to levels that existed in the early 20th century. A temperature rise of 1.5 degrees would reduce the area suitable for forests by 20% by the year 2050, because some tropical areas will become too hot. The countries that have the most forest-ready land are: Russia, Canada, Brazil, Australia, the United States and China.
Impacts on temperature are affected by the location of the forest. For example, reforestation in boreal or subarctic regions has less impact on climate. This is because it substitutes a high-albedo, snow-dominated region with a lower-albedo forest canopy. By contrast, tropical reforestation projects lead to a positive change such as the formation of clouds. These clouds then reflect the sunlight, lowering temperatures.
Planting trees in tropical climates with wet seasons has another advantage. In such a setting, trees grow more quickly (fixing more carbon) because they can grow year-round. Trees in tropical climates have, on average, larger, brighter, and more abundant leaves than non-tropical climates. A study of the girth of 70,000 trees across Africa has shown that tropical forests fix more carbon dioxide pollution than previously realized. The research suggested almost one fifth of fossil fuel emissions are absorbed by forests across Africa, Amazonia and Asia. Simon Lewis stated, "Tropical forest trees are absorbing about 18% of the carbon dioxide added to the atmosphere each year from burning fossil fuels, substantially buffering the rate of change."
A 2019 study of the global potential for tree restoration showed that there is space for at least 9 million km2 of new forests worldwide, which is a 25% increase from current conditions. This forested area could store up to 205 gigatons of carbon or 25% of the atmosphere's current carbon pool by reducing CO2 in the atmosphere.
Wetlands


Wetland restoration involves restoring a wetland's natural biological, geological, and chemical functions through re-establishment or rehabilitation. It is a good way to reduce climate change. Wetland soil, particularly in coastal wetlands such as mangroves, sea grasses, and salt marshes, is an important carbon reservoir; 20–30% of the world's soil carbon is found in wetlands, while only 5–8% of the world's land is composed of wetlands. Studies have shown that restored wetlands can become productive CO2 sinks and many are being restored. Aside from climate benefits, wetland restoration and conservation can help preserve biodiversity, improve water quality, and aid with flood control.
The plants that make up wetlands absorb carbon dioxide (CO2) from the atmosphere and convert it into organic matter. The waterlogged nature of the soil slows down the decomposition of organic material, leading to the accumulation of carbon-rich peat, acting as a long-term carbon sink. Also, anaerobic conditions in waterlogged soils hinder the complete breakdown of organic matter, promoting the conversion of carbon into more stable forms.
As with forests, for the sequestration process to succeed, the wetland must remain undisturbed. If it is disturbed the carbon stored in the plants and sediments will be released back into the atmosphere, and the ecosystem will no longer function as a carbon sink. Additionally, some wetlands can release non-CO2 greenhouse gases, such as methane and nitrous oxide which could offset potential climate benefits. The amounts of carbon sequestered via blue carbon by wetlands can also be difficult to measure.
Wetland soil is an important carbon sink; 14.5% of the world's soil carbon is found in wetlands, while only 5.5% of the world's land is composed of wetlands. Not only are wetlands a great carbon sink, they have many other benefits like collecting floodwater, filtering out air and water pollutants, and creating a home for numerous birds, fish, insects, and plants.
Climate change could alter wetland soil carbon storage, changing it from a sink to a source. With rising temperatures comes an increase in greenhouse gasses from wetlands especially locations with permafrost. When this permafrost melts it increases the available oxygen and water in the soil. Because of this, bacteria in the soil would create large amounts of carbon dioxide and methane to be released into the atmosphere.
The link between climate change and wetlands is still not fully known. It is also not clear how restored wetlands manage carbon while still being a contributing source of methane. However, preserving these areas would help prevent further release of carbon into the atmosphere.
Peatlands, mires and peat bogs
Peatlands hold approximately 30% of the carbon in our ecosystem. When they are drained for agricultural land and urbanization, because peatlands are so vast, large quantities of carbon decompose and emit CO2 into the atmosphere. The loss of one peatland could potentially produce more carbon than 175–500 years of methane emissions.
Peat bogs act as a sink for carbon because they accumulate partially decayed biomass that would otherwise continue to decay completely. There is a variance on how much the peatlands act as a carbon sink or carbon source that can be linked to varying climates in different areas of the world and different times of the year. By creating new bogs, or enhancing existing ones, the amount of carbon that is sequestered by bogs would increase.
Agriculture
Compared to natural vegetation, cropland soils are depleted in soil organic carbon (SOC). When soil is converted from natural land or semi-natural land, such as forests, woodlands, grasslands, steppes, and savannas, the SOC content in the soil reduces by about 30–40%. This loss is due to harvesting, as plants contain carbon. When land use changes, the carbon in the soil will either increase or decrease, and this change will continue until the soil reaches a new equilibrium. Deviations from this equilibrium can also be affected by variated climate.
The decreasing of SOC content can be counteracted by increasing the carbon input. This can be done with several strategies, e.g. leave harvest residues on the field, use manure as fertilizer, or include perennial crops in the rotation. Perennial crops have a larger below ground biomass fraction, which increases the SOC content.
Perennial crops reduce the need for tillage and thus help mitigate soil erosion, and may help increase soil organic matter. Globally, soils are estimated to contain >8,580 gigatons of organic carbon, about ten times the amount in the atmosphere and much more than in vegetation.
Researchers have found that rising temperatures can lead to population booms in soil microbes, converting stored carbon into carbon dioxide. In laboratory experiments heating soil, fungi-rich soils released less carbon dioxide than other soils.
Following carbon dioxide (CO2) absorption from the atmosphere, plants deposit organic matter into the soil. This organic matter, derived from decaying plant material and root systems, is rich in carbon compounds. Microorganisms in the soil break down this organic matter, and in the process, some of the carbon becomes further stabilized in the soil as humus - a process known as humification.
On a global basis, it is estimated that soil contains about 2,500 gigatons of carbon. This is greater than 3-fold the carbon found in the atmosphere and 4-fold of that found in living plants and animals. About 70% of the global soil organic carbon in non-permafrost areas is found in the deeper soil within the upper metre and is stabilized by mineral-organic associations.
Carbon farming
Carbon farming is a set of agricultural methods that aim to store carbon in the soil, crop roots, wood and leaves. The technical term for this is carbon sequestration. The overall goal of carbon farming is to create a net loss of carbon from the atmosphere. This is done by increasing the rate at which carbon is sequestered into soil and plant material. One option is to increase the soil's organic matter content. This can also aid plant growth, improve soil water retention capacity and reduce fertilizer use. Sustainable forest management is another tool that is used in carbon farming. Carbon farming is one component of climate-smart agriculture. It is also one way to remove carbon dioxide from the atmisphere.
Agricultural methods for carbon farming include adjusting how tillage and livestock grazing is done, using organic mulch or compost, working with biochar and terra preta, and changing the crop types. Methods used in forestry include reforestation and bamboo farming.
Carbon farming methods might have additional costs. Some countries have government policies that give financial incentives to farmers to use carbon farming methods. As of 2016, variants of carbon farming reached hundreds of millions of hectares globally, of the nearly 5 billion hectares (1.2×1010 acres) of world farmland. Carbon farming is not without its challenges or disadvantages. This is because some of its methods can affect ecosystem services. For example, carbon farming could cause an increase of land clearing, monocultures and biodiversity loss. It is important to maximize environmental benefits of carbon farming by keeping in mind ecosystem services at the same time.Prairies
Prairie restoration is a conservation effort to restore prairie lands that were destroyed due to industrial, agricultural, commercial, or residential development. The primary aim is to return areas and ecosystems to their previous state before their depletion. The mass of SOC able to be stored in these restored plots is typically greater than the previous crop, acting as a more effective carbon sink.
Biochar
Biochar is charcoal created by pyrolysis of biomass waste. The resulting material is added to a landfill or used as a soil improver to create terra preta.
Adding biochar may increase the soil-C stock for the long term and so
mitigate global warming by offsetting the atmospheric C (up to 9.5
Gigatons C annually). In the soil, the biochar carbon is unavailable for oxidation to CO
2
and consequential atmospheric release. However concerns have been
raised about biochar potentially accelerating release of the carbon
already present in the soil.
Terra preta, an anthropogenic, high-carbon soil, is also being investigated as a sequestration mechanism. By pyrolysing biomass, about half of its carbon can be reduced to charcoal, which can persist in the soil for centuries, and makes a useful soil amendment, especially in tropical soils (biochar or agrichar).
Burial of biomass

Burying biomass (such as trees) directly mimics the natural processes that created fossil fuels. The global potential for carbon sequestration using wood burial is estimated to be 10 ± 5 GtC/yr and largest rates in tropical forests (4.2 GtC/yr), followed by temperate (3.7 GtC/yr) and boreal forests (2.1 GtC/yr). In 2008, Ning Zeng of the University of Maryland estimated 65 GtC lying on the floor of the world's forests as coarse woody material which could be buried and costs for wood burial carbon sequestration run at 50 USD/tC which is much lower than carbon capture from e.g. power plant emissions. CO2 fixation into woody biomass is a natural process carried out through photosynthesis. This is a nature-based solution and methods being trialled include the use of "wood vaults" to store the wood-containing carbon under oxygen-free conditions.
In 2022 a certification organization published methodologies for biomass burial. Other biomass storage proposals have included the burial of biomass deep underwater, including at the bottom of the Black Sea.
Geological carbon sequestration
Underground storage in suitable geologic formations
Geological sequestration refers to the storage of CO2 underground in depleted oil and gas reservoirs, saline formations, or deep, coal beds unsuitable for mining.
Once CO2 is captured from a point source, such as a cement factory, it can be compressed to ≈100 bar into a supercritical fluid. In this form, the CO2 could be transported via pipeline to the place of storage. The CO2 could then be injected deep underground, typically around 1 km (0.6 mi), where it would be stable for hundreds to millions of years. Under these storage conditions, the density of supercritical CO2 is 600 to 800 kg/m3.
The important parameters in determining a good site for carbon storage are: rock porosity, rock permeability, absence of faults, and geometry of rock layers. The medium in which the CO2 is to be stored ideally has a high porosity and permeability, such as sandstone or limestone. Sandstone can have a permeability ranging from 1 to 10−5 Darcy, with a porosity as high as ≈30%. The porous rock must be capped by a layer of low permeability which acts as a seal, or caprock, for the CO2. Shale is an example of a very good caprock, with a permeability of 10−5 to 10−9 Darcy. Once injected, the CO2 plume will rise via buoyant forces, since it is less dense than its surroundings. Once it encounters a caprock, it will spread laterally until it encounters a gap. If there are fault planes near the injection zone, there is a possibility the CO2 could migrate along the fault to the surface, leaking into the atmosphere, which would be potentially dangerous to life in the surrounding area. Another risk related to carbon sequestration is induced seismicity. If the injection of CO2 creates pressures underground that are too high, the formation will fracture, potentially causing an earthquake.
Structural trapping is considered the principal storage mechanism, impermeable or low permeability rocks such as mudstone, anhydrite, halite, or tight carbonates act as a barrier to the upward buoyant migration of CO2, resulting in the retention of CO2 within a storage formation. While trapped in a rock formation, CO2 can be in the supercritical fluid phase or dissolve in groundwater/brine. It can also react with minerals in the geologic formation to become carbonates.
Mineral sequestration
Mineral sequestration aims to trap carbon in the form of solid carbonate salts. This process occurs slowly in nature and is responsible for the deposition and accumulation of limestone over geologic time. Carbonic acid in groundwater slowly reacts with complex silicates to dissolve calcium, magnesium, alkalis and silica and leave a residue of clay minerals. The dissolved calcium and magnesium react with bicarbonate to precipitate calcium and magnesium carbonates, a process that organisms use to make shells. When the organisms die, their shells are deposited as sediment and eventually turn into limestone. Limestones have accumulated over billions of years of geologic time and contain much of Earth's carbon. Ongoing research aims to speed up similar reactions involving alkali carbonates.
Zeolitic imidazolate frameworks
Zeolitic imidazolate frameworks (ZIFs) are metal–organic frameworks similar to zeolites. Because of their porosity, chemical stability and thermal resistance, ZIFs are being examined for their capacity to capture carbon dioxide.
Mineral carbonation
CO2 exothermically reacts with metal oxides, producing stable carbonates (e.g. calcite, magnesite). This process (CO2-to-stone) occurs naturally over periods of years and is responsible for much surface limestone. Olivine is one such metal oxide. Rocks rich in metal oxides that react with CO2, such as MgO and CaO as contained in basalts, have been proven as a viable means to achieve carbon-dioxide mineral storage. The reaction rate can in principle be accelerated with a catalyst or by increasing pressures, or by mineral pre-treatment, although this method can require additional energy. Formation of carbonates is considered to be the safest capturing mechanism of CO2.
Earthen oxide | Percent of crust | Carbonate | Enthalpy change (kJ/mol) |
---|---|---|---|
CaO | 4.90 | CaCO3 | −179 |
MgO | 4.36 | MgCO3 | −118 |
Na2O | 3.55 | Na2CO3 | −322 |
FeO | 3.52 | FeCO3 | −85 |
K2O | 2.80 | K2CO3 | −393.5 |
Fe2O3 | 2.63 | FeCO3 | 112 |
All oxides | 21.76 | All carbonates |
Ultramafic mine tailings are a readily available source of fine-grained metal oxides that could serve this purpose. Accelerating passive CO2 sequestration via mineral carbonation may be achieved through microbial processes that enhance mineral dissolution and carbonate precipitation.
Carbon, in the form of CO
2 can be removed from the atmosphere by chemical processes, and stored in stable carbonate mineral forms. This process (CO
2-to-stone) is known as "carbon sequestration by mineral carbonation" or mineral sequestration. The process involves reacting carbon dioxide with abundantly available metal oxides – either magnesium oxide (MgO) or calcium oxide (CaO) – to form stable carbonates. These reactions are exothermic and occur naturally (e.g., the weathering of rock over geologic time periods).
- CaO + CO
2 → CaCO
3
- MgO + CO
2 → MgCO
3
Calcium and magnesium are found in nature typically as calcium and magnesium silicates (such as forsterite and serpentinite) and not as binary oxides. For forsterite and serpentine the reactions are:
- Mg
2SiO
4 + 2 CO
2 → 2 MgCO
3 + SiO
2
- Mg
3Si
2O
5(OH)
4+ 3 CO
2 → 3 MgCO
3 + 2 SiO
2 + 2 H
2O
These reactions are slightly more favorable at low temperatures. This process occurs naturally over geologic time frames and is responsible for much of the Earth's surface limestone. The reaction rate can be made faster however, by reacting at higher temperatures and/or pressures, although this method requires some additional energy. Alternatively, the mineral could be milled to increase its surface area, and exposed to water and constant abrasion to remove the inert silica as could be achieved naturally by dumping olivine in the high energy surf of beaches. Experiments suggest the weathering process is reasonably quick (one year) given porous basaltic rocks.
The reaction yield, that is the amount of CO2 mineralized per unit mass of the target material, is rarely achieved as per stoichiometry and as such, higher temperature, pressure and even chemical reagents will have to be used to achieve a better yield in a short time. As mineralized products occupy more volume than the originally excavated rocks, the environmental impacts associated with landfilling more material than was excavated in the first place must be considered.
CO
2 naturally reacts with peridotite rock in surface exposures of ophiolites, notably in Oman. It has been suggested that this process can be enhanced to carry out natural mineralization of CO
2.[123][124]
When CO
2 is dissolved in water and injected into hot basaltic rocks underground it has been shown that the CO
2 reacts with the basalt to form solid carbonate minerals. A test plant in Iceland started up in October 2017, extracting up to 50 tons of CO2 a year from the atmosphere and storing it underground in basaltic rock.
Researchers from British Columbia developed a low cost process for the production of magnesite, also known as magnesium carbonate, which can sequester CO2 from the air, or at the point of air pollution, e.g. at a power plant. The crystals are naturally occurring, but accumulation is usually very slow.
Concrete is a promising destination for captured carbon dioxide. Several advantages that concrete offers include, but not limited to: a source of plenty of calcium due to its substantial production all over the world; a thermodynamically stable condition for carbon dioxide to be stored as calcium carbonates; and its long-term capability of storing carbon dioxide as a material widely used in infrastructure. Demolished concrete waste or recycled concrete could be also used aside from newly produced concrete. Studies at HeidelbergCement show that carbon sequestration can turn demolished and recycled concrete into a supplementary cementitious material, which can act as a secondary binder in tandem with Portland cement, in new concrete production.
Sequestration in oceans
Marine carbon pumps

The ocean naturally sequesters carbon through different processes. The solubility pump moves carbon dioxide from the atmosphere into the surface ocean where it reacts with water molecules to form carbonic acid. The solubility of carbon dioxide increases with decreasing water temperatures. Thermohaline circulation moves dissolved carbon dioxide to cooler waters where it is more soluble, increasing carbon concentrations in the ocean interior. The biological pump moves dissolved carbon dioxide from the surface ocean to the ocean's interior through the conversion of inorganic carbon to organic carbon by photosynthesis. Organic matter that survives respiration and remineralization can be transported through sinking particles and organism migration to the deep ocean.
The low temperatures, high pressure, and reduced oxygen levels in the deep sea slow down decomposition processes, preventing the rapid release of carbon back into the atmosphere and acting as a long-term storage reservoir.
Vegetated coastal ecosystems
Seaweed farming and algae

Seaweed grow in shallow and coastal areas, and capture significant amounts of carbon that can be transported to the deep ocean by oceanic mechanisms; seaweed reaching the deep ocean sequester carbon and prevent it from exchanging with the atmosphere over millennia. Growing seaweed offshore with the purpose of sinking the seaweed in the depths of the sea to sequester carbon has been suggested. In addition, seaweed grows very fast and can theoretically be harvested and processed to generate biomethane, via anaerobic digestion to generate electricity, via cogeneration/CHP or as a replacement for natural gas. One study suggested that if seaweed farms covered 9% of the ocean they could produce enough biomethane to supply Earth's equivalent demand for fossil fuel energy, remove 53 gigatonnes of CO2 per year from the atmosphere and sustainably produce 200 kg per year of fish, per person, for 10 billion people. Ideal species for such farming and conversion include Laminaria digitata, Fucus serratus and Saccharina latissima.
Both macroalgae and microalgae are being investigated as possible means of carbon sequestration. Marine phytoplankton perform half of the global photosynthetic CO2 fixation (net global primary production of ~50 Pg C per year) and half of the oxygen production despite amounting to only ~1% of global plant biomass.
Because algae lack the complex lignin associated with terrestrial plants, the carbon in algae is released into the atmosphere more rapidly than carbon captured on land. Algae have been proposed as a short-term storage pool of carbon that can be used as a feedstock for the production of various biogenic fuels.

Large-scale seaweed farming could sequester huge amounts of carbon. Wild seaweed will sequester large amount of carbon through dissolved particles of organic matter being transported to deep ocean seafloors where it will become buried and remain for long periods of time. Currently seaweed farming is carried out to provide food, medicine and biofuel. In respect to carbon farming, the potential growth of seaweed for carbon farming would see the harvested seaweed transported to the deep ocean for long-term burial. Seaweed farming has gathered attention given the limited terrestrial space available for carbon farming practices. Currently seaweed farming occurs mostly in the Asian Pacific coastal areas where it has been a rapidly increasing market. The IPCC Special Report on the Ocean and Cryosphere in a Changing Climate recommends "further research attention" on seaweed farming as a mitigation tactic.
However, seaweed farming, and carbon farming in general, only keeps the carbon within the fast carbon cycle, in intimate contact with the ocean and atmosphere, and once in equilibrium with the ecology, cannot be expected to hold additional carbon.
Ocean fertilization

Ocean fertilization or ocean nourishment is a type of technology for carbon dioxide removal from the ocean based on the purposeful introduction of plant nutrients to the upper ocean to increase marine food production and to remove carbon dioxide from the atmosphere. Ocean nutrient fertilization, for example iron fertilization, could stimulate photosynthesis in phytoplankton. The phytoplankton would convert the ocean's dissolved carbon dioxide into carbohydrate, some of which would sink into the deeper ocean before oxidizing. More than a dozen open-sea experiments confirmed that adding iron to the ocean increases photosynthesis in phytoplankton by up to 30 times.
This is one of the more well-researched carbon dioxide removal (CDR) approaches, however this approach would only sequester carbon on a timescale of 10-100 years dependent on ocean mixing times. While surface ocean acidity may decrease as a result of nutrient fertilization, when the sinking organic matter remineralizes, deep ocean acidity will increase. A 2021 report on CDR indicates that there is medium-high confidence that the technique could be efficient and scalable at low cost, with medium environmental risks. One of the key risks of nutrient fertilization is nutrient robbing, a process by which excess nutrients used in one location for enhanced primary productivity, as in a fertilization context, are then unavailable for normal productivity downstream. This could result in ecosystem impacts far outside the original site of fertilization.
A number of techniques, including fertilization by the micronutrient iron (called iron fertilization) or with nitrogen and phosphorus (both macronutrients), have been proposed. But research in the early 2020s suggested that it could only permanently sequester a small amount of carbon.Artificial upwelling
Artificial
upwelling or downwelling is an approach that would change the mixing
layers of the ocean. Encouraging various ocean layers to mix can move
nutrients and dissolved gases around. Mixing may be achieved by placing large vertical pipes in the oceans to pump nutrient rich water to the surface, triggering blooms of algae, which store carbon when they grow and export carbon when they die. This produces results somewhat similar to iron fertilization. One side-effect is a short-term rise in CO
2, which limits its attractiveness.
Mixing layers involve transporting the denser and colder deep ocean water to the surface mixed layer. As the ocean temperature decreases with depth, more carbon dioxide and other compounds are able to dissolve in the deeper layers. This can be induced by reversing the oceanic carbon cycle through the use of large vertical pipes serving as ocean pumps, or a mixer array. When the nutrient rich deep ocean water is moved to the surface, algae bloom occurs, resulting in a decrease in carbon dioxide due to carbon intake from phytoplankton and other photosynthetic eukaryotic organisms. The transfer of heat between the layers will also cause seawater from the mixed layer to sink and absorb more carbon dioxide. This method has not gained much traction as algae bloom harms marine ecosystems by blocking sunlight and releasing harmful toxins into the ocean. The sudden increase in carbon dioxide on the surface level will also temporarily decrease the pH of the seawater, impairing the growth of coral reefs. The production of carbonic acid through the dissolution of carbon dioxide in seawater hinders marine biogenic calcification and causes major disruptions to the oceanic food chain.
Basalt storage
Carbon dioxide sequestration in basalt involves the injecting of CO
2 into deep-sea formations. The CO
2
first mixes with seawater and then reacts with the basalt, both of
which are alkaline-rich elements. This reaction results in the release
of Ca2+ and Mg2+ ions forming stable carbonate minerals.
Underwater basalt offers a good alternative to other forms of
oceanic carbon storage because it has a number of trapping measures to
ensure added protection against leakage. These measures include
"geochemical, sediment, gravitational and hydrate formation." Because CO
2 hydrate is denser than CO
2 in seawater, the risk of leakage is minimal. Injecting the CO
2 at depths greater than 2,700 meters (8,900 ft) ensures that the CO
2 has a greater density than seawater, causing it to sink.
One possible injection site is Juan de Fuca plate. Researchers at the Lamont–Doherty Earth Observatory found that this plate at the western coast of the United States has a possible storage capacity of 208 gigatons. This could cover the entire current U.S. carbon emissions for over 100 years.
This process is undergoing tests as part of the CarbFix project, resulting in 95% of the injected 250 tonnes of CO2 to solidify into calcite in two years, using 25 tonnes of water per tonne of CO2.
Mineralization and deep sea sediments
Similar to mineralization processes that take place within rocks, mineralization can also occur under the sea. The rate of dissolution of carbon dioxide from atmosphere to oceanic regions is determined by the circulation period of the ocean and buffering ability of subducting surface water. Researchers have demonstrated that the carbon dioxide marine storage at several kilometers depth could be viable for up to 500 years, but is dependent on injection site and conditions. Several studies have shown that although it may fix carbon dioxide effectively, carbon dioxide may be released back to the atmosphere over time. However, this is unlikely for at least a few more centuries. The neutralization of CaCO3, or balancing the concentration of CaCO3 on the seafloor, land and in the ocean, can be measured on a timescale of thousands of years. More specifically, the predicted time is 1700 years for ocean and approximately 5000 to 6000 years for land. Further, the dissolution time for CaCO3 can be improved by injecting near or downstream of the storage site.
In addition to carbon mineralization, another proposal is deep sea sediment injection. It injects liquid carbon dioxide at least 3,000 m (9,800 ft) below the surface directly into ocean sediments to generate carbon dioxide hydrate. Two regions are defined for exploration: 1) the negative buoyancy zone (NBZ), which is the region between liquid carbon dioxide denser than surrounding water and where liquid carbon dioxide has neutral buoyancy, and 2) the hydrate formation zone (HFZ), which typically has low temperatures and high pressures. Several research models have shown that the optimal depth of injection requires consideration of intrinsic permeability and any changes in liquid carbon dioxide permeability for optimal storage. The formation of hydrates decreases liquid carbon dioxide permeability, and injection below HFZ is more energetically favored than within the HFZ. If the NBZ is a greater column of water than the HFZ, the injection should happen below the HFZ and directly to the NBZ. In this case, liquid carbon dioxide will sink to the NBZ and be stored below the buoyancy and hydrate cap. Carbon dioxide leakage can occur if there is dissolution into pore fluid or via molecular diffusion. However, this occurs over thousands of years.
Adding bases to neutralize acids
Carbon dioxide forms carbonic acid when dissolved in water, so ocean acidification
is a significant consequence of elevated carbon dioxide levels, and
limits the rate at which it can be absorbed into the ocean (the solubility pump). A variety of different bases have been suggested that could neutralize the acid and thus increase CO
2 absorption. For example, adding crushed limestone to oceans enhances the absorption of carbon dioxide. Another approach is to add sodium hydroxide to oceans which is produced by electrolysis of salt water or brine, while eliminating the waste hydrochloric acid by reaction with a volcanic silicate rock such as enstatite, effectively increasing the rate of natural weathering of these rocks to restore ocean pH.
Single-step carbon sequestration and storage
Single-step carbon sequestration and storage is a saline water-based mineralization technology extracting carbon dioxide from seawater and storing it in the form of solid minerals.
Abandoned ideas
Direct deep-sea carbon dioxide injection
It was once suggested that CO2 could be stored in the oceans by direct injection into the deep ocean and storing it there for some centuries. At the time, this proposal was called "ocean storage" but more precisely it was known as "direct deep-sea carbon dioxide injection". However, the interest in this avenue of carbon storage has much reduced since about 2001 because of concerns about the unknown impacts on marine life , high costs and concerns about its stability or permanence. The "IPCC Special Report on Carbon Dioxide Capture and Storage" in 2005 did include this technology as an option. However, the IPCC Fifth Assessment Report in 2014 no longer mentioned the term "ocean storage" in its report on climate change mitigation methods. The most recent IPCC Sixth Assessment Report in 2022 also no longer includes any mention of "ocean storage" in its "Carbon Dioxide Removal taxonomy".
Applications in climate change policies
United States
The Executive Order on Tackling the Climate Crisis at Home and Abroad, signed by president Joe Biden on January 27, 2021, includes several mentions of carbon sequestration via conservation and restoration of carbon sink ecosystems, such as wetlands and forests. These include emphasizing the importance of farmers, landowners, and coastal communities in carbon sequestration, directing the Treasury Department to promote conservation of carbon sinks through market based mechanisms, and directing the Department of the Interior to collaborate with other agencies to create a Civilian Climate Corps to increase carbon sequestration in agriculture, among other things.