Sketch of nuclear thermal rocket
1 December 1967: The first ground experimental nuclear rocket engine
(XE) assembly is shown here in "cold flow" configuration, as it makes a
late evening arrival at Engine Test Stand No. 1 at the Nuclear Rocket
Development Station in Jackass Flats, Nevada.
A nuclear thermal rocket is a proposed spacecraft propulsion technology. In a nuclear thermal rocket a working fluid, usually liquid hydrogen, is heated to a high temperature in a nuclear reactor, and then expands through a rocket nozzle to create thrust. In this kind of thermal rocket, the nuclear reactor's energy replaces the chemical energy of the propellant's reactive chemicals in a chemical rocket. The thermal heater / inert propellant paradigm as opposed to the reactive propellants of chemical rockets turns out to produce a superior effective exhaust velocity, and therefore a superior propulsive efficiency, with specific impulses on the order of twice that of chemical engines. The overall gross lift-off mass of a nuclear rocket is about half that of a chemical rocket, and hence when used as an upper stage it roughly doubles or triples the payload carried to orbit.[citation needed]
A nuclear engine was considered for some time as a replacement for the J-2 used on the S-II and S-IVB stages on the Saturn V and Saturn I rockets. Originally "drop-in" replacements were considered for higher performance, but a larger replacement for the S-IVB stage was later studied for missions to Mars and other high-load profiles, known as the S-N. Nuclear thermal space tugs were originally planned as one component of the Space Transportation System, taking payloads from low Earth orbit to higher orbits, the Moon, and other planets. Robert Bussard proposed the single-stage-to-orbit "Aspen" vehicle using a nuclear thermal rocket for propulsion and liquid hydrogen propellant for partial shielding against neutron back scattering in the lower atmosphere.[1] The Soviet Union studied nuclear engines for their own moon rockets, notably upper stages of the N-1, although they never entered an extensive testing program like the one the U.S. conducted throughout the 1960s at the Nevada Test Site. Despite many successful firings, American nuclear rockets did not fly before the space race ended.
To date, no nuclear thermal rocket has flown, although the NERVA NRX/EST and NRX/XE were built and tested with flight design components. The highly successful U.S. Project Rover which ran from 1955 through 1972 accumulated over 17 hours of run time. The NERVA NRX/XE, judged by SNPO to be the last "technology development" reactor necessary before proceeding to flight prototypes, accumulated over 2 hours of run time, including 28 minutes at full power.[2] The Russian nuclear thermal rocket RD-0410 was also claimed by the Soviets to have gone through a series of tests at the nuclear test site near Semipalatinsk.[3][4]
The United States tested twenty different sizes and designs during Project Rover and NASA's NERVA program from 1959 through 1972 at the Nevada Test Site, designated Kiwi, Phoebus, NRX/EST, NRX/XE, Pewee, Pewee 2 and the Nuclear Furnace, with progressively higher power densities culminating in the Pewee (1970) and Pewee 2.[2] Tests of the improved Pewee 2 design were cancelled in 1970 in favor of the lower-cost Nuclear Furnace (NF-1), and the U.S. nuclear rocket program officially ended in spring of 1973. Current (2010) 110 kN (25,000 lbf) reference designs (NERVA-Derivative Rockets, or NDRs) are based on the Pewee, and have specific impulses of 925 seconds.[citation needed]
Types
A nuclear thermal rocket can be categorized by the construction of its reactor, which can range from a relatively simple solid reactor up to a much more complicated but more efficient reactor with a gas core. As with all thermal rocket designs, the specific impulse produced is proportional to the square root of the temperature to which the working fluid (reaction mass) is heated, and hence the most efficient designs require the highest temperatures possible. This is typically limited by the properties of materials.Solid core
The most traditional type uses a conventional (albeit light-weight) nuclear reactor running at high temperatures to heat the working fluid that is moving through the reactor core. This is known as the solid-core design, and is the simplest design to construct.
A NERVA solid-core design
Bimodal Nuclear Thermal Rocket in Low Earth Orbit (artistic rendering)
A solid core reactor's performance is ultimately limited by the melting point of the materials used in the reactor cores. The design needs to be constructed of materials that remain strong at as high a temperature as possible, as nuclear reactions can create much higher temperatures than most materials can typically withstand, meaning that much of the potential of the reactor for very high temperatures might not be realized. Additionally problematic, since there is no cooling medium at work (as in an Earth bound pressurized water reactor), is the cracking of fuel rod coatings due to the large temperature ranges they would experience (from 22 K up to 3000 K over the length of a 1.3 m fuel rod), and the necessity of matching coefficients of expansion in all the components of the reactor. Using hydrogen as a propellant, a solid core design would typically deliver specific impulses (Isp) on the order of 850 to 1000 seconds, which is about twice that of liquid hydrogen-oxygen designs such as the Space Shuttle main engine. Other propellants have also been proposed, such as ammonia, water or LOX, and while these propellants would provide reduced exhaust velocity (and, hence, performance) when compared to hydrogen, their generally greater availability could reduce payload costs by a very large factor, and perform adequately where the mission delta-v is not too high (such as within cislunar space or between Earth orbit and Martian orbit). Still, yet another mark in favor of hydrogen is that above about 1500 K, it begins to dissociate at low pressures, or around 3000 K at high pressures, which is a potential area of promise for increasing the Isp of solid core reactors.
The weight of a solid core reactor was initially thought to be its main drawback. Immediately after World War II, a complete nuclear reactor was so heavy that it was feared that solid core nuclear thermal engines would be hard-pressed [5] to achieve a thrust-to-weight ratio of 1:1, which is needed to overcome the gravity of the Earth at launch. This problem was quickly overcome,[how?] however, and over the next twenty-five years U.S. nuclear thermal rocket designs eventually reached thrust-to-weight ratios of approximately 7:1. This is still a much lower thrust-to-weight ratio than what is achievable with chemical rockets, which have thrust-to-weight ratios on the order of 70:1. That fact, when combined with the large tanks necessary for liquid hydrogen (or other working fluid) storage, means that solid core nuclear thermal engines are best suited for a) use in upper stages, where vehicle velocity is already near orbital, or b) in space "tugs", or intra Solar System transports, which would be used to take payloads and/or people between gravity wells, or c) for launches from a planet smaller than Earth, a planetoid (or "dwarf planet"), an asteroid, or a moon where the required thrust is lower due to lower gravity fields. Currently then, for a solid core nuclear thermal rocket to be more useful than chemical rockets as a first or second stage Earth launch engine, the system would either have to be made much lighter, or provide even higher specific impulse. Until that day, it can be said[by whom?] that the true strength of nuclear rockets currently lies in solar system exploration, outside Earth's gravity well.
One way to increase the temperature, and thus the specific impulse, is to isolate the nuclear fuel elements so they no longer have to be of the rigid fuel rod type. This is the basis of the particle-bed reactor, also known as the fluidized-bed, dust-bed, or rotating-bed design. In this design the fuel is placed in a number of (typically spherical) elements which "float" inside the hydrogen working fluid. Spinning the entire engine forces the fuel elements out to walls that are being cooled by the hydrogen. This design increases the specific impulse to about 1000 seconds (9.8 kN·s/kg), allowing for thrust-to-weight ratios greater than 1:1, although at the cost of increased complexity. Such a design could share design elements with a pebble-bed reactor, several of which are currently generating electricity.
From 1987 through 1991, the SDI Office funded Project Timberwind, a non-rotating nuclear thermal rocket based on particle bed technology. Although the project was canceled before testing in 1992 by the incoming Clinton Administration, the design was thought to achieve thrust-to-weight ratios of 30:1 and specific impulses of at least 1000 seconds.
Pulsed nuclear thermal rocket
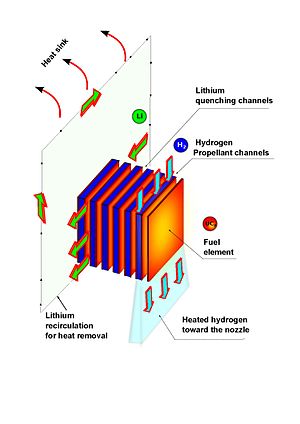
Pulsed nuclear thermal rocket unit cell concept for Isp
amplification. In this cell, hydrogen-propellant is heated by the
continuous intense neutron pulses in the propellant channels. At the
same time, the unwanted energy from the fission fragments is removed by a
solitary cooling channel with lithium or other liquid metal.
The pulsed nuclear thermal rocket, (not to be confused with nuclear pulse propulsion which is a hypothetical method of spacecraft propulsion that uses nuclear explosions for thrust), is a type of solid nuclear thermal rocket for thrust and specific impulse (Isp) amplification.[6] In this concept, the conventional solid NTR can operate in a stationary as well as in a pulsed mode -much like a TRIGA reactor. Because the residence time of the propellant in the chamber is short, an important amplification in energy is attainable by pulsing the nuclear core which can increase the thrust via increasing the propellant mass flow. However, the most interesting feature is the capability to obtain very high propellant temperatures (higher than the fuel) and then high amplification of exhaust velocity. This is because, in contrast with the convectional stationary solid NTR, propellant is heated by the intense neutron flux from the pulsation, which is directly transported from the fuel to the propellant as kinetic energy, by pulsing the core it is possible to obtain a propellant hotter than the fuel. However, and in clear contrast with classical nuclear thermal rockets (including liquid and gas nuclear rockets), the energy from[clarification needed] fission fragments are unwanted.
Very high instantaneous propellant temperatures are hypothetically attainable by pulsing the solid nuclear core, only limited by the rapid radiative cooling after pulsation.
Liquid core
Dramatically greater improvements are theoretically possible by mixing the nuclear fuel into the working fluid and allowing the reaction to take place in the liquid mixture itself. This idea is the basis of the liquid-core engine which can operate at temperatures above the melting point of the nuclear fuel; the maximum operating temperature of the engine is determined by the maximum temperature that the container wall (typically a neutron reflector of some sort) can withstand while it is actively cooled by the hydrogen. The liquid-core design is expected to deliver specific impulse performance on the order of 1300 to 1500 seconds (12.8–14.8 kN·s/kg).These engines are currently considered to be very difficult to build. The reaction time of the nuclear fuel is much longer than the heating time of the working fluid and therefore requires a method to trap the fuel inside the engine while allowing the working fluid to easily exit through the nozzle. Most liquid-phase engines have focused on rotating the fuel/fluid mixture at very high speeds to force the fuel to the outside by centripetal force (uranium is more dense than hydrogen). The design mirrors the particle-bed design in many ways but operates at even higher temperatures.
Robert Zubrin has proposed an alternative liquid-core design, the nuclear salt-water rocket. In this design, water is the working fluid and also serves as the neutron moderator. The nuclear fuel is not retained which drastically simplifies the design. However, by its very design, the rocket would discharge massive quantities of extremely radioactive waste and could only be safely operated well outside the Earth's atmosphere and perhaps even entirely outside earth's magnetosphere.
Gas core
Nuclear gas core closed cycle rocket engine diagram, nuclear "light bulb"
Nuclear gas core open cycle rocket engine diagram
The final classification is the gas-core engine. This is a modification to the liquid-core design which uses rapid circulation of the fluid to create a toroidal pocket of gaseous uranium fuel in the middle of the reactor, surrounded by hydrogen. In this case the fuel does not touch the reactor wall at all, so temperatures could reach several tens of thousands of degrees, which would allow specific impulses of 3000 to 5000 seconds (30 to 50 kN·s/kg). In this basic design, the "open cycle", the losses of nuclear fuel would be difficult to control, which has led to studies of the "closed cycle" or nuclear lightbulb engine, where the gaseous nuclear fuel is contained in a super-high-temperature quartz container, over which the hydrogen flows. The closed cycle engine actually has much more in common with the solid-core design, but this time is limited by the critical temperature of quartz instead of the fuel stack. Although less efficient than the open-cycle design, the closed-cycle design is expected to deliver a specific impulse of about 1500–2000 seconds (15–20 kN·s/kg).
History
The KIWI A prime nuclear thermal rocket engine
Although engineering studies of all of these designs were made, only the solid-core engine was ever built. Development of such engines started under the aegis of the Atomic Energy Commission in 1955 as Project Rover, with work on a suitable reactor starting at Los Alamos National Laboratory and Area 25 in the Nevada Test Site. Four basic designs came from this project: KIWI, Phoebus, Pewee and the Nuclear Furnace. Twenty rockets were tested.
When NASA was formed in 1958, it was given authority over all non-nuclear aspects of the Rover program. In order for NASA to cooperate with the AEC, the Space Nuclear Propulsion Office was created at the same time. In 1961, the NERVA program (Nuclear Engine for Rocket Vehicle Applications) was created. Marshall Space Flight Center had been increasingly using KIWI for mission planning, and NERVA was formed to formalize the entry of nuclear thermal rocket engines into space exploration. Unlike the AEC work, which was intended to study the reactor design itself, NERVA's goal was to produce a real engine that could be deployed on space missions. A 75,000 lbf (334 kN) thrust baseline NERVA design was based on the KIWI B4 series, and was considered for some time as the upper stages for the Saturn V, in place of the J-2s that were actually flown.
Although the Kiwi/Phoebus/NERVA designs were the only ones to be tested in any substantial program, a number of other solid-core engines were also studied to some degree. The Small Nuclear Rocket Engine, or SNRE, was designed at the Los Alamos National Laboratory (LANL) for upper stage use, both on unmanned launchers as well as the Space Shuttle. It featured a split-nozzle that could be rotated to the side, allowing it to take up less room in the Shuttle cargo bay. The design provided 73 kN of thrust and operated at a specific impulse of 875 seconds (8.58 kN·s/kg), and it was planned to increase this to 975 with fairly basic upgrades. This allowed it to achieve a mass fraction of about 0.74, comparing with 0.86 for the SSME, one of the best conventional engines.
A related design that saw some work, but never made it to the prototype stage, was Dumbo. Dumbo was similar to KIWI/NERVA in concept, but used more advanced construction techniques to lower the weight of the reactor. The Dumbo reactor consisted of several large barrel-like tubes which were in turn constructed of stacked plates of corrugated material. The corrugations were lined up so that the resulting stack had channels running from the inside to the outside. Some of these channels were filled with uranium fuel, others with a moderator, and some were left open as a gas channel. Hydrogen was pumped into the middle of the tube, and would be heated by the fuel as it travelled through the channels as it worked its way to the outside. The resulting system was lighter than a conventional design for any particular amount of fuel. The project developed some initial reactor designs and appeared to be feasible.[citation needed]
Between 1987 and 1991 an advanced engine design was studied under Project Timberwind, under the aegis of the Strategic Defense Initiative ("Star Wars"), which was later expanded into a larger design in the Space Thermal Nuclear Propulsion (STNP) program. Advances in high-temperature metals, computer modelling and nuclear engineering in general resulted in dramatically improved performance. While the NERVA engine was projected to weigh about 6,803 kg, the final STNP offered just over 1/3 the thrust from an engine of only 1,650 kg by improving the Isp to between 930 and 1000 seconds.[citation needed]
In January 2012, the propulsion group for Project Icarus began a technology development project, known as Project Bifrost, under the auspices of Icarus Interstellar and General Propulsion Sciences, to develop an NTR propulsion system, initially aimed at interplanetary missions.[7]
Test firings
KIWI was the first to be fired, starting in July 1959 with KIWI 1. The reactor was not intended for flight, hence the naming of the rocket after a flightless bird. This was unlike later tests because the engine design could not really be used; the core was simply a stack of uncoated uranium oxide plates onto which the hydrogen was dumped. Nevertheless, it generated 70 MW and produced an exhaust temperature of 2683 K. Two additional tests of the basic concept, A1 and A3, added coatings to the plates to test fuel rod concepts.The KIWI B series fully developed the fuel system, which consisted of the uranium fuel in the form of tiny uranium dioxide (UO2) spheres embedded in a low-boron graphite matrix, and then coated with niobium carbide. Nineteen holes ran the length of the bundles, and through these holes the liquid hydrogen flowed for cooling. A final change introduced during the KIWI program changed the fuel to uranium carbide, which was run for the last time in 1964.
On the initial firings immense reactor heat and vibration cracked the fuel bundles. Likewise, while the graphite materials used in the reactor's construction were indeed resistant to high temperatures, they eroded under the heat and pressure of the enormous stream of superheated hydrogen. The fuel bundle problem was largely (but not completely) solved by the end of the program, and related materials work at Argonne National Laboratory looked promising. Fuel and engine coatings never wholly solved this problem before the program ended.
Building on the KIWI series, the Phoebus series were much larger reactors. The first 1A test in June 1965 ran for over 10 minutes at 1090 MW, with an exhaust temperature of 2370 K. The B run in February 1967 improved this to 1500 MW for 30 minutes. The final 2A test in June 1968 ran for over 12 minutes at 4,000 MW, at the time the most powerful nuclear reactor ever built.
NERVA NRX (Nuclear Rocket Experimental), started testing in September 1964. The final engine in this series was the XE, designed with flight design hardware and fired in a downward position into a low-pressure chamber to simulate a vacuum. SNPO fired NERVA NRX/XE twenty-eight times in March 1968. The series all generated 1100 MW, and many of the tests concluded only when the test-stand ran out of hydrogen propellant. NERVA NRX/XE produced the baseline 75,000 lbf (334 kN) thrust that Marshall required in Mars mission plans.
A KIWI engine being destructively tested
A smaller version of KIWI, the Pewee was also built. It was fired several times at 500 MW in order to test coatings made of zirconium carbide (instead of niobium carbide) but Pewee also increased the power density of the system. An unrelated water-cooled system known as NF-1 (for Nuclear Furnace) was used for future materials testing. Pewee became the basis for current NTR designs being researched at NASA's Glenn and Marshall Research Centers.
The last NRX firing lost a relatively small 17 kilograms (38 lb) of fuel in 2 hours of testing, enough to be judged sufficient for space missions by SNPO. Pewee 2's fuel elements reduced fuel corrosion still further, by a factor of 3 in Nuclear Furnace testing, but Pewee 2 was never tested on the stand. Later designs were deemed by NASA to be usable for space exploration and Los Alamos felt that it had cured the last of the materials problems with the untested Pewee.
The NERVA/Rover project was eventually cancelled in 1972 with the general wind-down of NASA in the post-Apollo era. Without a manned mission to Mars, the need for a nuclear thermal rocket was unclear. Another perceived problem would be an intense public outcry against any attempt to use a nuclear engine.
Nuclear vs. chemical
Directly comparing the performance of a nuclear engine and a chemical one is not easy; the design of any rocket is a study in compromises and different ideas of what constitutes "better". The outline considers the NERVA-derived engine proposed by NASA in the 1960s, comparing it with the S-IVB stage from the Saturn it was intended to replace.For any given thrust, the amount of power that needs to be generated is defined by
However, as outlined above, even the simple solid-core design provided a large increase in Isp to about 850 seconds. Using the formula above, we can calculate the amount of power that needs to be generated, at least given extremely efficient heat transfer: P = (1014 kN)(850 s × 9.81 m/s2)/2 = 4,227 MW. The Isp improvement demands higher energy. Given inefficiencies in the heat transfer, the actual NERVA designs were planned to produce about 5 GW, which would make them the most powerful nuclear reactors in the world.
The fuel flow for any given thrust level can be found from
Finally, one must consider the design of the stage as a whole. The S-IVB carried just over 300,000 litres of fuel; 229,000 litres of liquid hydrogen (17,300 kg), and 72,700 litres of liquid oxygen (86,600 kg). The S-IVB uses a common bulkhead between the tanks, so removing it to produce a single larger tank would increase the total load only slightly. A new hydrogen-only nuclear stage would thus carry just over 300,000 litres in total (300 m³), or about 21,300 kg (47,000 lb). At 1,725 litres per second, this is a burn time of only 175 seconds, compared to about 500 in the original S-IVB (although some of this is at a lower power setting).
The total change in velocity, the so-called delta-v, can be found from the rocket equation, which is based on the starting and ending masses of the stage:
Following the formula above, this means the J-2 powered version generates a Δv of (414 s × 9.81) ln(119,900/13,311), or 8,900 m/s. The nuclear-powered version assumed above would be (850×9.81) ln(38,600/17,300), or 6,700 m/s. This drop in overall performance is due largely to the much higher "burnout" weight of the engine, and to smaller burn time due to the less-dense fuel. As a drop-in replacement, then, the nuclear engine does not seem to offer any advantages.
However, this simple examination ignores several important issues. For one, the new stage weighs considerably less than the older one, which means that the lower stages below it will leave the new upper stage at a higher velocity. This alone will make up for much of the difference in performance. More importantly, the comparison assumes that the stage would otherwise remain the same design overall. This is a bad assumption; one generally makes the upper stages as large as they can be given the throw-weight of the stages below them. In this case one would not make a drop-in version of the S-IVB, but a larger stage whose overall weight was the same as the S-IVB.
Following that line of reasoning, we can envision a replacement S-IVB stage that weighs 119,900 kg fully fueled, which would require much larger tanks. Assuming that the tankage mass triples, we have a m1 of 31,500 + 6,800 = 38,300 kg, and since we have fixed
Of course this increase in tankage might not be easy to arrange. NASA actually considered a new S-IVB replacement, the S-N, built to be as physically large as possible while still being able to be built in the VAB. It weighed only 10,429 kg empty and 53,694 kg fueled (suggesting that structural loading is the dominant factor in stage mass, not the tankage). The combination of lower weight and higher performance improved the payload of the Saturn V as a whole from 127,000 kg delivered to low earth orbit (LEO) to 155,000 kg.
It is also worth considering the improvement in stage performance using the more advanced engine from the SNTP program. Using the same S-IVB baseline, which does make sense in this case due to the lower thrust, we have an unfueled weight (
In summation, NASA's current reasoning is that a NPR can be developed that would be twice as efficient as its chemical counterpart, though it is likely such an engine would only be used beyond the Earth's atmosphere.
Risks
An atmospheric or orbital rocket failure could result in the dispersal of radioactive material into the environment. A collision with orbital debris, material failure due to uncontrolled fission, material imperfections or fatigue, or human design flaws could cause a containment breach of the fissile material. Such a catastrophic failure while in flight could release radioactive material over the Earth in a wide and unpredictable area. The amount of contamination would depend on the size of the nuclear thermal rocket engine, while the zone of contamination and its concentration would be dependent on prevailing weather conditions and orbital parameters at the time of re-entry.It is unlikely that a reactor's fuel elements would be spread over a wide area because the fuel elements in solid-core nuclear thermal rockets are designed to withstand very high temperatures (up to 3500K) and high pressures (up to 200 atm): they are composed of very strong materials such as carbon composites or carbides and are normally coated with zirconium hydride. Conventionally, the solid core NTR fuel itself is a small percentage of U-235 buried well inside an extremely strong carbon or carbide mixture. The radioactivity of these elements is quite low and would pose a minimal hazard unless the physically small reactors had been run for an extended period.
Kiwi-TNT
In January 1965, the U.S. Rover program purposely placed a Kiwi Reactor (KIWI-TNT) on fast excursion to simulate a worst-case scenario of a fall from altitude into the ocean such as might occur in a booster failure after launch. The rocket was positioned on a railroad car in the Jackass Flats area of the Nevada Test Site, with the reactor specially modified so as to go prompt critical.The radiation released would have caused fatalities out to 600 feet and injuries out to 2000 feet.[8]
Current solid-core nuclear thermal rocket designs greatly limit the dispersion and break-up of radioactive fuel elements.[9] This is a result of the many open-air nuclear weapons tests that were conducted in the 1950s.[10]
Current research
At its Marshall Space Flight Center, NASA is (in 2013) simulating nuclear thermal rocket fuels with the interim goal of developing a Nuclear Cryogenic Propulsion Stage in support of the Space Launch System. The project could see rocket stages twice as efficient as their chemical counterparts propelling crewed missions to the Moon, Mars and beyond.[11]On March 1, 2018 Russia's president Vladimir Putin claimed that at the end of 2017 Russia had successfully tested a nuclear-powered cruise missile. The nuclear engine mounted on the missile was described by Putin as "compact and heavy-duty" and the missile itself as having "practically unlimited range" and an unpredictable flight path to avoid U.S. missile defense systems.[12]