Original link: https://www.edge.org/conversation/the-inflationary-universe-alan-guth
Inflationary theory itself is a twist on the conventional Big Bang theory. The shortcoming that inflation is intended to fill in is the basic fact that although the Big Bang theory is called the Big Bang theory it is, in fact, not really a theory of a bang at all; it never was.
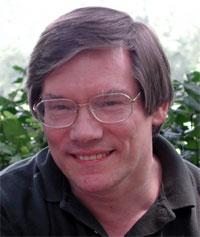
ALAN GUTH, father of the inflationary theory of the Universe, is Victor F. Weisskopf Professor of Physics at MIT; author of The Inflationary Universe: The Quest for a New Theory of Cosmic Origins.
THE INFLATIONARY UNIVERSE: ALAN GUTH
[ALAN GUTH:] Paul Steinhardt did a very good job of presenting the case for the cyclic universe. I'm going to describe the conventional consensus model upon which he was trying to say that the cyclic model is an improvement. I agree with what Paul said at the end of his talk about comparing these two models; it is yet to be seen which one works. But there are two grounds for comparing them. One is that in both cases the theory needs to be better developed. This is more true for the cyclic model, where one has the issue of what happens when branes collide. The cyclic theory could die when that problem finally gets solved definitively. Secondly, there is, of course, the observational comparison of the gravitational wave predictions of the two models.
A brane is short for membrane, a term that comes out of string theories. String theories began purely as theories of strings, but when people began to study their dynamics more carefully, they discovered that for consistency it was not possible to have a theory which only discussed strings. Whereas a string is a one-dimensional object, the theory also had to include the possibility of membranes of various dimensions to make it consistent, which led to the notion of branes in general. The theory that Paul described in particular involves a four-dimensional space plus one time dimension, which he called the bulk. That four-dimensional space was sandwiched between two branes.
That's not what I'm going to talk about. I want to talk about the conventional inflationary picture, and in particular the great boost that this picture has attained over the past few years by the somewhat shocking revelation of a new form of energy that exists in the universe. This energy, for lack of a better name, is typically called "dark energy."
But let me start the story further back. Inflationary theory itself is a twist on the conventional Big Bang theory. The shortcoming that inflation is intended to overcome is the basic fact that, although the Big Bang theory is called the Big Bang it is in fact not really a theory of a bang at all; it never was. The conventional Big Bang theory, without inflation, was really only a theory of the aftermath of the Bang. It started with all of the matter in the universe already in place, already undergoing rapid expansion, already incredibly hot. There was no explanation of how it got that way. Inflation is an attempt to answer that question, to say what "banged," and what drove the universe into this period of enormous expansion. Inflation does that very wonderfully. It explains not only what caused the universe to expand, but also the origin of essentially all the matter in the universe at the same time. I qualify that with the word "essentially" because in a typical version of the theory inflation needs about a gram's worth of matter to start. So, inflation is not quite a theory of the ultimate beginning, but it is a theory of evolution that explains essentially everything that we see around us, starting from almost nothing.
The basic idea behind inflation is that a repulsive form of gravity caused the universe to expand. General relativity from its inception predicted the possibility of repulsive gravity; in the context of general relativity you basically need a material with a negative pressure to create repulsive gravity. According to general relativity it's not just matter densities or energy densities that create gravitational fields; it's also pressures. A positive pressure creates a normal attractive gravitational field of the kind that we're accustomed to, but a negative pressure would create a repulsive kind of gravity. It also turns out that according to modern particle theories, materials with a negative pressure are easy to construct out of fields which exist according to these theories. By putting together these two ideas — the fact that particle physics gives us states with negative pressures, and that general relativity tells us that those states cause a gravitational repulsion — we reach the origin of the inflationary theory.
By answering the question of what drove the universe into expansion, the inflationary theory can also answer some questions about that expansion that would otherwise be very mysterious. There are two very important properties of our observed universe that were never really explained by the Big Bang theory; they were just part of one's assumptions about the initial conditions. One of them is the uniformity of the universe — the fact that it looks the same everywhere, no matter which way you look, as long as you average over large enough volumes. It's both isotropic, meaning the same in all directions, and homogeneous, meaning the same in all places. The conventional Big Bang theory never really had an explanation for that; it just had to be assumed from the start. The problem is that, although we know that any set of objects will approach a uniform temperature if they are allowed to sit for a long time, the early universe evolved so quickly that there was not enough time for this to happen. To explain, for example, how the universe could have smoothed itself out to achieve the uniformity of temperature that we observe today in the cosmic background radiation, one finds that in the context of the standard Big Bang theory, it would be necessary for energy and information to be transmitted across the universe at about a hundred times the speed of light.
In the inflationary theory this problem goes away completely, because in contrast to the conventional theory it postulates a period of accelerated expansion while this repulsive gravity is taking place. That means that if we follow our universe backwards in time towards the beginning using inflationary theory, we see that it started from something much smaller than you ever could have imagined in the context of conventional cosmology without inflation. While the region that would evolve to become our universe was incredibly small, there was plenty of time for it to reach a uniform temperature, just like a cup of coffee sitting on the table cools down to room temperature. Once this uniformity is established on this tiny scale by normal thermal-equilibrium processes — and I'm talking now about something that's about a billion times smaller than the size of a single proton — inflation can take over, and cause this tiny region to expand rapidly, and to become large enough to encompass the entire visible universe. The inflationary theory not only allows the possibility for the universe to be uniform, but also tells us why it's uniform: It's uniform because it came from something that had time to become uniform, and was then stretched by the process of inflation.
The second peculiar feature of our universe that inflation does a wonderful job of explaining, and for which there never was a prior explanation, is the flatness of the universe — the fact that the geometry of the universe is so close to Euclidean. In the context of relativity, Euclidean geometry is not the norm; it's an oddity. With general relativity, curved space is the generic case. In the case of the universe as a whole, once we assume that the universe is homogeneous and isotropic, then this issue of flatness becomes directly related to the relationship between the mass density and the expansion rate of the universe. A large mass density would cause space to curve into a closed universe in the shape of a ball; if the mass density dominated, the universe would be a closed space with a finite volume and no edge. If a spaceship traveled in what it thought was a straight line for a long enough distance, it would end up back where it started from. In the alternative case, if the expansion dominated, the universe would be geometrically open. Geometrically open spaces have the opposite geometric properties from closed spaces. They're infinite. In a closed space two lines which are parallel will start to converge; in an open space two lines which are parallel will start to diverge. In either case what you see is very different from Euclidean geometry. However, if the mass density is right at the borderline of these two cases, then the geometry is Euclidean, just like we all learned about in high school.
In terms of the evolution of the universe, the fact that the universe is at least approximately flat today requires that the early universe was extraordinarily flat. The universe tends to evolve away from flatness, so even given what we knew ten or twenty years ago — we know much better now that the universe is extraordinarily close to flat — we could have extrapolated backwards and discovered that, for example, at one second after the Big Bang the mass density of the universe must have been equal, to an accuracy of 15 decimal places, to the critical density where it counterbalanced the expansion rate to produce a flat universe. The conventional Big Bang theory gave us no reason to believe that there was any mechanism to require that, but it has to have been that way to explain why the universe looks the way it does today. The conventional Big Bang theory without inflation really only worked if you fed into it initial conditions which were highly finely tuned to make it just right to produce a universe like the one we see. Inflationary theory gets around this flatness problem because inflation changes the way the geometry of the universe evolves with time. Even though the universe always evolves away from flatness at all other periods in the history of the universe, during the inflationary period the universe is actually driven towards flatness incredibly quickly. If you had approximately 10-34 seconds or so of inflation at the beginning of the universe, that's all you need to be able to start out a factor of 105 or 1010 away from being flat. Inflation would then have driven the universe to be flat closely enough to explain what we see today.
There are two primary predictions that come out of inflationary models that appear to be testable today. They have to do (1) with the mass density of the universe, and (2) with the properties of the density non-uniformities. I'd like to say a few words about each of them, one at a time. Let me begin with the question of flatness.
The mechanism that inflation provides that drives the universe towards flatness will in almost all cases overshoot, not giving us a universe that is just nearly flat today, but a universe that's almost exactly flat today. This can be avoided, and people have at times tried to design versions of inflation that avoided it, but these versions of inflation never looked very plausible. You have to arrange for inflation to end at just the right point, where it's almost made the universe flat but not quite. It requires a lot of delicate fine-tuning, but in the days when it looked like the universe was open some people tried to design such models. But they always looked very contrived, and never really caught on.
The generic inflationary model drives the universe to be completely flat, which means that one of the predictions is that today the mass density of the universe should be at the critical value which makes the universe geometrically flat. Until three or four years ago no astronomers believed that. They told us that if you looked at just the visible matter, you would see only about one percent of what you needed to make the universe flat. But they also said that they could offer more than that — there's also dark matter. Dark matter is matter that's inferred to exist because of the gravitational effect that it has on visible matter. It's seen, for example, in the rotation curves of galaxies. When astronomers first measured how fast galaxies rotate, they found they were spinning so fast that if the only matter present was what you saw, galaxies would just fly apart.
To understand the stability of galaxies it was necessary to assume that there was a large amount of dark matter in the galaxy — about five or ten times the amount of visible matter — which was needed just to hold the galaxy together. This problem repeats itself when one talks about the motion of galaxies within clusters of galaxies. The motion of galaxies in clusters is much more random and chaotic than the spiral galaxy, but the same issues arise. You can ask how much mass is needed to hold those clusters of galaxies together, and the answer is that you still need significantly more matter than what you assumed was in the galaxies. Adding all of that together, astronomers came up only to about a third of the critical density. They were pretty well able to guarantee that there wasn't any more than that out there; that was all they could detect. That was bad for the inflationary model, but many of us still had faith that inflation had to be right and that sooner or later the astronomers would come up with something.
And they did, although what they came up with was something very different from the kind of matter that we were talking about previously. Starting in 1998, astronomers have been gathering evidence for the remarkable fact that the universe today appears to be accelerating, not slowing down. As I said at the beginning of this talk, the theory of general relativity allows for that. What's needed is a material with a negative pressure. We are now therefore convinced that our universe must be permeated with a material with negative pressure, which is causing the acceleration that we're now seeing. We don't know what this material is, but we're referring to it as "dark energy." Even without knowing what it is, general relativity by itself allows us to calculate how much mass has to be out there to cause the observed acceleration, and it turns out to be almost exactly equal to two-thirds of the critical density. This is exactly what was missing from the previous calculations! So, if we assume that this dark energy is real, we now have complete agreement between what the astronomers are telling us about the mass density of the universe and what inflation predicts.
The other important prediction that comes out of inflation is becoming even more persuasive than the issue of flatness: namely, the issue of density perturbations. Inflation has what in some ways is a wonderful characteristic — that by stretching everything out (and Paul's model takes advantage of the same effect) you can smooth out any non-uniformities that were present prior to this expansion. Inflation does not depend sensitively on what you assume existed before inflation; everything there just gets washed away by the enormous expansion. For a while, in the early days of developing the inflationary model, we were all very worried that this would lead to a universe that would be absolutely, completely smooth. After a while several physicists began to explore the idea that quantum fluctuations could save us. The universe is fundamentally a quantum mechanical system, so perhaps quantum theory was necessary not just to understand atoms, but also to understand galaxies. It is a rather remarkable idea that an aspect of fundamental physics like quantum theory could have such a broad sweep. The point is that a classical version of inflationary theory would predict a completely uniform density of matter at the end of inflation. According to quantum mechanics, however, everything is probabilistic. There are quantum fluctuations everywhere, which means that in some places the mass density would be slightly higher than average, and in other places it would be slightly lower than average. That's exactly the sort of thing you want to explain the structure of the universe. You can even go ahead and calculate the spectrum of these non-uniformities, which is something that Paul and I both worked on in the early days and had great fun with. The answer that we both came up with was that, in fact, quantum mechanics produces just the right spectrum of non-uniformities.
We really can't predict the overall amplitude — that is, the intensity of these ripples — unless we know more about the fundamental theory. At the present time, we have to take the overall factor that multiplies the predicted intensity of these ripples from observation. But we can predict the spectrum — that is, the complicated pattern of ripples can be viewed as ripples of many different wavelengths lying on top of each other, and we can calculate how the intensity of the ripples varies with their wavelengths. We knew how to do this back in 1982, but recently it has actually become possible for astronomers to see these non-uniformities imprinted on the cosmic background radiation. These were first observed back in 1992 by the COBE (Cosmic Background Explorer) satellite, but back then they could only see very broad features, since the angular resolution of the satellite was only about seven degrees. Now, they've gotten down to angular resolutions of about a tenth of a degree. These observations of the cosmic background radiation can be used to produce plots of the spectrum of non-uniformities, which are becoming more and more detailed.
The most recent data set was made by an experiment called the Cosmic Background Imager, which released a new set of data in May that is rather spectacular. This graph of the spectrum is rather complicated because these fluctuations are produced during the inflationary era, but then oscillate as the early universe evolves. Thus, what you see is a picture that includes the original spectrum plus all of the oscillations which depend on various properties of the universe. A remarkable thing is that these curves now show five separate peaks, and all five of the peaks show good agreement between theory and observation. You can see that the peaks are in about the right place and have about the right heights, without any ambiguity, and the leading peak is rather well-mapped-out. It's a rather remarkable fit between actual measurements made by astronomers, and a theory based on wild ideas about quantum fluctuations at 10-35 seconds. The data is so far in beautiful agreement with the theory.
At the present time this inflationary theory, which a few years ago was in significant conflict with observation now works perfectly with our measurements of the mass density and the fluctuations. The evidence for a theory that's either the one that I'm talking about or something very close to it is very, very strong.
I'd just like to close by saying that although I've been using the theory in the singular to talk about inflation I shouldn't, really. It's very important to remember that inflation is really a class of theories. If inflation is right it's by no means the end of our study of the origin of the universe, but still, it's really closer to the beginning. There are many different versions of inflation, and in fact the cyclic model that Paul described could be considered one version. It's a rather novel version since it puts the inflation at a completely different era of the history of the universe, but inflation is still doing many of the same things. There are many versions of inflation that are much closer to the kinds of theories that we were developing in the '80s and '90s, so saying that inflation is right is by no means the end of the story. There's still a lot of flexibility here, and a lot to be learned. And what needs to be learned will involve both the study of cosmology and the study of the underlying particle physics, which is essential to these models.